Verdict in Neurodegeneration: Murder by Amyloid Protofibrils
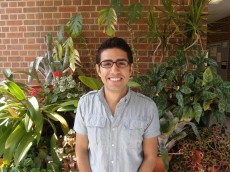
Jaime Perez
Download PDF
Neurodegenerative diseases are devastating human disorders characterized by neuronal cell death. Each disease is linked to the misfolding of specific proteins that accumulate and cause intracellular or extracellular brain inclusions. However, the mechanism by which neuronal death develops is still unknown. Since these protein aggregates are typically fibrillar, one hypothesis identifies such fibrils as neurotoxic. My lab primarily focused on biochemical analysis of misfolded and aggregated proteins in Prion disease, Alzheimer’s disease (AD), and Parkinson’s disease (PD). In Prion disease, we developed in vitro assays that recapitulated how a protease-resistant Prion form of the PrP protein causes the change in shape of the healthy form, leading to dangerous accumulation of the toxic, infectious Prion shape. In AD and PD, we discovered prefibrillar oligomers, which we named protofibrils. We believe this to be the true toxic conformer, as one of its properties is to permeabilize lipid membranes. In contrast, the fibrillar aggregates are likely protective. We have also investigated how polymorphisms in an ubiquitin hydrolase (UCHL-1) involved in PD can be either protective or a risk factor. Thus, our insight into protein misfolding of diverse proteins provides new avenues for developing treatments and cures against these neurodegenerative diseases.
Introduction
Our society is afflicted by a variety of human brain disorders. Amongst them are a group of disorders classified as neurodegenerative diseases. Neurodegeneration involves the deterioration or death of neurons in the brain, specific to the disease. This degeneration is devastating because regeneration in the central nervous system is extremely restricted1.
Neurodegenerative diseases affect millions of people worldwide and unfortunately, no cure currently exists. Understanding the molecular and cellular mechanisms by which these diseases operate, might eventually lead to more effective treatments and ultimately a cure. By 2040, neurodegenerative diseases will surpass cancer as one of the leading causes of death2. The effect on the human population provides the necessary motivation to study their pathogenesis.
The best-studied neurodegenerative diseases include, Alzheimer’s disease (AD), Lou Gehrig’s disease, Creutzfeldt-Jacob disease, Huntington disease, Parkinson’s disease (PD), and Transmissible Spongiform Encephalopathies (TSEs), or Prion diseases. These all vary in symptoms and time of onset, but one common and shared characteristic is the presence of an abnormal protein accumulation unique to each disease. In each specific disease, a particular protein misfolds, aggregates, and usually leads to accumulation as intracellular or extracellular inclusions in the brain of patients3. These inclusions are often fibrillar in nature4. In most cases, these proteins are thought to gain a toxic new function, leading to pathogenesis, but data is not yet conclusive.
This review focuses on studies that have attempted to provide a relationship between protein aggregation and neuronal cell death. We focus on three neurodegenerative diseases: Prion disease, AD, and PD. Studies with humans are difficult because symptoms do not arise until later in the disease, but some studies with animals have proven to be effective. My lab, however, focuses on characterizing the early stages of this protein aggregation process, by specifically isolating the aggregates from post- mortem brains and performing kinetic studies of in vitro protein aggregation4. Additionally, by understanding the in vitro effects of the protein aggregates, we can establish a link between aggregation and toxicity5.
We have advanced the understanding of protein aggregates by characterizing a special case of proteins called prions (here on referred to as PrP). These are different from other aggregation prone proteins in that they are transmissible. A protease-resistant Prion form of PrP causes the change in shape of the healthy form, leading to dangerous accumulations of the toxic infectious prion shape6.
We also have contributed to the field by discovering prefibrillar intermediates in AD and PD, referred here as protofibrils, which are precursors to amyloid formation. We also believe these are the true toxic species conformer, because one of their properties is to permeabilize lipid membranes. In contrast, the fibrillar aggregates are likely protective7,8. Moreover, we have also investigated how polymorphisms in an ubiquitin hydrolase (UCHL-1) involved in PD can either provide protection or increase risk for the onset of the disease9.
Prion Disease
In my lab, we first characterized Prion disease and the Prion protein (PrP) as the culprit protein. Prion diseases include scrapie, bovine spongiform encephalopathy (BSE), or more commonly known as mad cow disease, and human Creutzfeldt-Jakob disease (CJD). Prion diseases are different than other neurodegenerative diseases because they are transmissible across individuals of one species, and in certain cases, between different species as well4. Symptoms of Prion diseases vary, but holes in post-mortem brains remain to be the hallmark symptom10.
The Unique Case of the Prion Protein
The question of which protein accumulates in Prion diseased brains was puzzling. Recently, PrP was determined to have more than one conformation, and one of these conformations were found in individuals with Prion disease, linking it as a possible disease-causing shape. Non-disease causing PrP (here on referred to as PrPc) is usually a protease-sensitive protein. What is interesting is that the possible disease-causing PrP (here on referred to as PrPsc) is resistant to proteases. Proteases or proteinases are enzymes that break down proteins by hydrolyzing thepeptide bonds that link amino acids together. A gap in the field was whether or not Prpsc converted Prpc into Prpsc.
Figure 1: Shape change of PrPC by PrPSC
In this model, PrPC (light gray circle) is interacting with PrPSC under certain cellular conditions; the interaction will cause a conformation change in PrPC to the PrPSC shape. The semi-circle structure at the top right of the light gray circle and the five dark gray squares demonstrate the conformation change from one state to another.
My lab was the first to report this conversion in vivo. Using this cell-free system was effective because the reactions occured quickly and we could use PrPsc from infected brains and PrPc from uninfected tissue11. We started the reaction with PrPsc from infected hamster brains and added radioactively labeled PrPc. After incubation for two days, protease was added and radioactively labeled PrP exhibited fragments of comparable size to infected PrPsc sources. These bands were not observed when PrPsc was not present, but was observed when PrPsc was highly denatured (but not completely). In fact, partial denaturation of PrPsc was optimal for conversion. Therefore, PrPc converted into PrPsc only when the latter is present, even at small amounts, which was demonstrated by determining its sensitivity to protease after the interaction6 (Figure 1).
Furthermore, the mechanism by which normal PrPc is converted into the protease-resistant PrPsc depends on a variety of factors. Firstly, increasing the concentration of PrPsc always increases the conversion rate of PrPc, which also increases with time. Secondly, in an experiment where PrPsc was centrifuged and the pellet and supernatant were tested for converting ability, we showed that the converting ability was only apparent in the pellet. This coincides with the idea that conversion is accelerated by aggregates12.
We further analyzed the species selectivity of Prion diseases, since this was an important question for understanding the basis of species barriers in transmission. We used PrPc and mouse, hamster and chimeric PrPsc to study this barrier. Results demonstrated that mouse PrPsc effectively converts hamster PrPc. Interestingly, mouse PrPc did not convert to protease-resistant PrPsc when hamster PrPsc was present. We further studied the primary sequence of PrPc to determine the conversion regions. Using mouse and hamster PrPc chimeras, we observed an area in the PrP sequence that seems to be critical in PrPsc species interaction, especially since mouse and hamster PrP sequences differ at residues 139, 155, and 17013.
Analyzing species-specificity of transmission is relevant since the infectious protein has the ability to cross species barriers. This is important when analyzing the infectivity to humans from eating infected meat from cows, as was demonstrated in the 1986 British BSE epidemic10. We provided evidence that the efficacy of transmission is much greater for homologous conversions than for cross- species conversions. Thus the ability of the infectious agent in BSE to affect humans is very low. The experiment tested the conversion rate of PrPc of and among human, sheep, mouse, hamster and bovine PrPsc, and little to no reaction was seen in non-homologous PrP combinations14.
Lastly, beyond species selectivity of PrP transmission, my lab has demonstrated the varying conversion of PrPc from different PrPsc strains. This was first observed in mice with drowsy and hyper strains, because their PrPsc were cleaved at different sites with protease. We demonstrated that when PrPc is taken from infected tissue and put into the culture with PrPc (of either strain), the PrPc converts into two distinct forms of PrPsc. This conversion is dependent upon what PrPsc strain is present. This suggests that there are different scrapie strains and pre-existing PrPsc can determine the conversion shape of PrPc 15.
My years of research with PrP have provided evidence of the necessity of PrPsc to be present in order for PrPc to be converted in a cell-free system, identifying a relationship to Prion disease infectivity. This work could further our understanding of cell death and protein aggregation in pathogenesis. Now, let’s examine AD and PD and how my lab discovered an intermediate in fibril formation that may be leading to cell death.
Alzheimer’s Disease
Continuing to identify the toxic agents in neurodegenerative diseases is important, and our work with AD has furthered our understanding of these culprit proteins. AD is the most prevalent neurodegenerative disease and is characterized by the loss of cognitive function4. Brains infected with AD are characterized by the presence of extracellular fibrillar amyloid plaques and intraneuronal neurofibrillary tangles. This is exhibited in the memory and learning brain areas. The plaques are mostly composed of the amyloid β-protein (Aβ), which is derived from the amyloid precursor protein (APP)16. Secretase enzymes break down Aβ in three ways: a harmless Aβ1-40, and two toxic Aβ1-42 and Aβ1-43 cuts17. Currently, data remains inconclusive about whether the presence of the protein will lead to pathogenesis.
Much research has been done to understand the Aβ protein. Fibrillar amyloid has been shown through X-ray diffraction data to contain the cross- β conformation. Prior to my work, one of the questions in the field was to determine the factors that lead to aggregation of the Aβ protein at the molecular level18. Therefore, we first focused on addressing the role of the hydrophobic C-terminal region of the protein in the stabilization and formation of fibrils. Conformational properties as well as solubility studies demonstrated that the C-terminal sequence, in particular the β 34-32 sequence, possesses a stable anti-parallel β-sheet. This is responsible for the insolubility of the β-protein, which also could implicate its role in amyloid plaque deposition18, 19.
Furthermore, the peptide NAC (non- Aβ component of AD) was identified as a component of the amyloid plaque, comprising of 10% of protein concentration. NAC is a fragment of α-synuclein, a protein involved at the pre-synaptic nerve terminal. Since this fragment is associated with AD plaques, it was necessary to understand its mechanism in amyloid formation. My lab answered this question by demonstrating that NAC amyloid can seed Aβprotein fibril formation and that Aβ protein and PrP can seed NAC amyloid.
Figure 2: Disease and protofibril formation may have a common cause
A number of factors including oxidative stress, aging, protein overexpression, pathogenic mutations, and impaired autophagy or proteasome, have been proposed to trigger protofibril formation. We propose a strong correlation between protofibril formation and neurodegenerative disease in patients’ brains. On the other hand, fibrils have been discussed as having a possible protective component.
This links the NAC precursor, part of α- synuclein, to neurodegeneration20. By further analyzing this possibly important component in amyloid deposition, we see that NACP is representative of a class of “natively unfolded” proteins from its unusual conformation properties. These proteins often have abilities in regulating protein-to-protein interactions21.
One important characteristic of Aβ is its location. In order to further understand Aβ, we wanted to test where it is mostly found within the brain. We demonstrate that the compartment in which Aβ resides in is a detergent-insoluble glycolipid membrane (DIG). This finding was important because it might be possible that APP makes the Aβ cuts there22.
Additionally, the apolipoprotein E (ApoE) allele is one risk factor for the development of late onset AD, because it is also involved in amyloid formation. Depending on the ApoE genotype, there is a strong correlation between it and the level of amyloid deposition. For example, ApoE4 is correlated with most deposition. My lab demonstrated that neither ApoE3 nor ApoE4 inhibited the seeded growth of amyloid fibrils. Therefore, when looking at inhibitors of amyloid formation it is important to examine the ApoE alleles23.
The Identification of a New Toxic Conformer
Now that fibril formation was further understood, the field now wanted to test if inhibition of the formation of amyloid fibrils had a therapeutic benefit. This would only be the case if fibril formation is toxic. If the pathogenic species were actually a precursor or an alternate to fibril formation, inhibiting fibrils would only increase toxicity. Therefore, it was essential to examine the mechanisms of fibril assembly and determine the true pathogenic species. Using atomic force microscopy (AFM) my lab was able to identify an intermediate in fibril formation, which we termed protofibril. AFM is a powerful technique because it has been used to determine early events in the process and is able to provide 10-20 nanometer resolutions of a particular species. Data demonstrates that Aβ protofibrils become longer and less numerous over time. Moreover, protofibrils disappear upon fibril formation. The elongation of protofibrils is also dependent on concentration7. Once these fibril intermediates were discovered, the question that still puzzled the field is if they are the true pathogenic species, which my lab strongly supports.
Lashuel et al. (2003) examined a mutation of the amyloid β-protein (Artic variant-E22G), demonstrating that Aβ40ARC accelerates fibril formation but also promotes the formation of annular protofibrils. Although the mechanism of protofibrillar toxicity is unknown, annular protofibrils have been implicated in forming ion-permeable pores in other neurodegenerative diseases. Therefore, membrane disruption by pore formation may be a causal factor that leads cell death24. Finally, to understand the role of protofibrils, we characterized their secondary structural features. We found that protofibrils had a very stable H- bonded core structure, possibly demonstrating their role in amyloid formation25 (Figure 2).
The work with AD performed in my lab has furthered the field’s understanding of Aβ in addition to the existence of the fibrillar intermediates named protofibrils, which have been implicated in amyloid formation. Further work with PD and protofibrils has increased our knowledge of the link between protofibrils and cell death, which is our next area of discussion.
Parkinson’s Disease
PD has furthered our understanding of the protofibril hypothesis in protein aggregation and it has been my latest area of focus in recent years. Classic PD symptoms include tremors, muscular rigidity, slowness of movement, and impaired balance and coordination. Similar to other neurodegenerative diseases, PD is linked to the misfolding and accumulation of the protein, α -synuclein, into structures called Lewy bodies located in the substantia nigra region of the midbrain. These individuals experience loss of dopaminergic neurons2,26,27,28,29. PD, like, AD also forms fibrils. Studies in my lab have demonstrated that mutant forms of α-synuclein (A30P and E46K) also form Lewy body- like fibrils in vitro30. Work with PD and α-synuclein has also demonstrated that α-synuclein forms anti-parallel β-sheets with fibrils, just like Aβ. Interestingly, researchers also saw protofibrils, but in a “sphere” shape. Overall, the data in the field links α-synuclein characteristics to other amyloid proteins31,32. Cytoplasmic concentrations of dopamine have been demonstrated to promote and stabilize protofibrillar intermediates, linking the dopaminergic selectivity of α- synuclein33.
Another radical hypothesis in the field is that synuclein membrane binding causes cell toxicity, as opposed to fibrillization in yeast. Studies show that α- synuclein disrupts normal membrane processes and eventually leads to toxicity31. Further work with yeast has demonstrated that the N-terminus has an important role in α- synuclein’s normal function. Therefore, its deletion causes toxicity.
Confirmation of the Protofibril Testimony
In order to answer the question of whether protofibrils are pathogenic or not, my lab continued to assess protofibril characteristics. Volles et al. (2001) provided clearer evidence of protofibrillar effects on cells. Data demonstrated that these protofibrillar intermediates binded tightly to synthetic vesicles via their β-sheet structure. This process causes a transient permeabilization of membranes, a potentially toxic event. This event could also lead to cellular stress and possibly apoptosis4. With this study, we have made a stronger case for the protofibrillar toxic hypothesis. Surprisingly, we have demonstrated that α-synuclein protofibrils did not bind to plasma membranes nor permeabilize synthetic vesicles. Ultimately, α-synuclein inhibits the protofibril and fibril formation of A53T, one of α- synuclein’s familial gene mutations36.
Examining USCH-L1
One important question that my lab has dealt with are the α- synuclein degradation routes. This is important because altered degradation of α synuclein has been implicated in the pathogenesis of PD. Data demonstrates that WT α-synuclein is degraded and internalized by the cell through chaperone- mediated autophagy (CMA)37. Dopamine-modified α- synuclein, on the other hand, has been demonstrated in my lab to be poorly degraded by CMA. Furthermore, this activity also blocks degradation of other common CMA substrates. Since an important degradation pathway is compromised, we saw an increase in cellular stressors. The link between dopamine modified α-synuclein and deficient CMA explains the selective degradation of dopaminergic cells38.
Further work with PD and degradation routes led my lab to studies involving an ubiquitin C-terminal hydrolase- L1 (UCH-L1), an enzyme that is critical for protein degradation. An interesting characteristic of UCH-L1 mutants is their ability to have both a protective component (S18Y), and to be a risk factor for the onset of the disease (I93M). My lab’s results demonstrated that the UCH-L1 mutation increases PD susceptibility through ligase activity9. UCH-L1 is also interesting because its hydrolase activity is tightly regulated. This allows it to only be active upon binding with a specific substrate, which induces a shape change39. Past data had suggested a role of UCH-L1 in proteasome activity. Liu et al. (2009) demonstrated that UCH-L1 exists in membrane-associated forms, which were enhanced by farnesylation. Moreover, this study demonstrated that UCH- L1 has no effect on the proteasome, perhaps linking lysosome degradation involvement. More importantly, farnesyltransferase inhibitors reduce α-synuclein levels and increase cell viability40.
Conclusion
It’s important to study neurodegenerative diseases because of their affliction on the human population. One characteristic shared by all diseases is the presence of an abnormally misfolded protein, and consequential cell death in a specific area of the brain. In most cases, these proteins are thought to gain a toxic new function, leading to pathogenesis, but data is not yet conclusive. My lab focuses on in vitro studies of PrP , Aβ, and α-synuclein.
Overall, we have characterized the shape change of the healthy PrP form to the protease-resistant form through interactions with the protease-resistant form, which, leads to pathogenesis. Furthermore, we discovered fibrillar intermediates, or protofibrils, which we believe are the true toxic species in amyloid formation. Their role has been associated with membrane permeabilization.
A limitation in our study is that we only dealt with assessing the molecular environment in vitro, which is not representative of other factors present in organisms or live cells. Understanding the role of amyloid formation in neurodegenerative diseases is a worldwide concern and need.
Acknowledgements
I would first like to thank Dr. Shubhik DebBurman for his guidance in understanding the literature in the field. I would also like to thank Mike Fiske for the writing feedback and everyone in BIOL 346 for their support.
Note: Eukaryon is published by students at Lake Forest College, who are solely responsible for its content. The views expressed in Eukaryon do not necessarily reflect those of the College. Articles published within Eukaryon should not be cited in bibliographies. Material contained herein should be treated as personal communication and should be cited as such only with the consent of the author.
References
1. Purves, D. (2008). Neuroscience (Sunderland, MA: Sinauer).
2. Lozano, A.M., and Kalia, S.K. (2005). New movements in Parkinson’s. Scientific American. 68-75
3. Taylor, J.P., Hardy, J., and Fischbeck, K.H. (2002). Toxic proteins in neurodegenerative disease. Science 296, 1991-1995.
4. Caughey, B., and Lansbury, P.T. (2003). Protofibrils, pores, fibrils, and neurodegeneration: separating the responsible protein aggregates from the innocent bystanders. Annu. Rev. Neurosci. 26, 267-298.
5. Lashuel, H.A., and Lansbury, P.T.,Jr. (2006). Are amyloid diseases caused by protein aggregates that mimic bacterial pore-forming toxins? Q. Rev. Biophys. 39, 167- 201.
6. Kocisko, D.A., Come, J.H., Priola, S.A., Chesebro, B., Raymond, G.J., Lansbury, P.T., and Caughey, B. (1994). Cell-free formation of protease-resistant prion protein. Nature 370, 471-474.
7. Harper, J.D., Wong, S.S., Lieber, C.M., and Lansbury, P.T.,Jr. (1999). Assembly of A beta amyloid protofibrils: an in vitro model for a possible early event in Alzheimer’s disease. Biochemistry 38, 8972- 8980.
8. Volles, M.J., and Lansbury, P.T.,Jr. (2002). Vesicle permeabilization by protofibrillar alpha-synuclein is sensitive to Parkinson’s disease-linked mutations and occurs by a pore-like mechanism. Biochemistry 41, 4595-4602.
9. Liu, Y., Fallon, L., Lashuel, H.A., Liu, Z., and Lansbury, P.T.,Jr. (2002). The UCH-L1 gene encodes two opposing enzymatic activities that affect alpha-synuclein degradation and Parkinson’s disease susceptibility. Cell 111, 209-218.
10. Prusiner, S.B. (1995). The prion diseases. Sci. Am. 272, 48-51, 54-7.
11. Caughey, B., Raymond, G.J., Priola, S.A., Kocisko, D.A., Race, R.E., Bessen, R.A., Lansbury, P.T.,Jr, and Chesebro, B. (1999). Methods for studying prion protein (PrP) metabolism and the formation of protease-resistant PrP in cell culture and cell-free systems. An update. Mol. Biotechnol. 13, 45-55.
12. Caughey, B., Kocisko, D.A., Raymond, G.J., and Lansbury, P.T.,Jr. (1995). Aggregates of scrapie-associated prion protein induce the cell-free conversion of protease-sensitive prion protein to the protease-resistant state. Chem. Biol. 2, 807-817.
13. Kocisko, D.A., Priola, S.A., Raymond, G.J., Chesebro, B., Lansbury, P.T.,Jr, and Caughey, B. (1995). Species specificity in the cell-free conversion of prion protein to protease-resistant forms: a model for the scrapie species barrier. Proc.Natl. Acad. Sci. U. S. A. 92, 3923-3927.
14. Raymond, G.J., Hope, J., Kocisko, D.A., Priola, S.A., Raymond, L.D., Bossers, A., Ironside, J., Will, R.G., Chen, S.G., Petersen, R.B., et al. (1997). Molecular assessment of the potential transmissibilities of BSE and scrapie to humans. Nature 388, 285- 288.
15. Bessen, R.A., Kocisko, D.A., Raymond, G.J., Nandan, S., Lansbury, P.T., and Caughey, B. (1995). Non-genetic propagation of strain-specific properties of scrapie prion protein. Nature 375, 698- 700.
16. Wolfe, M.S. (2006). Shutting down Alzheimer’s. Sci. Am. 294, 72- 79
17. St George-Hyslop, P.H. (2000). Piecing together Alzheimer’s. Sci. Am. 283, 76-83.
18. Halverson, K., Fraser, P.E., Kirschner, D.A., and Lansbury, P.T.,Jr. (1990). Molecular determinants of amyloid deposition in Alzheimer’s disease: conformational studies of synthetic beta-protein fragments. Biochemistry 29, 2639-2644.
19. Lansbury, P.T.,Jr, Costa, P.R., Griffiths, J.M., Simon, E.J., Auger, M., Halverson, K.J., Kocisko, D.A., Hendsch, Z.S., Ashburn, T.T., and Spencer, R.G. (1995). Structural model for the beta-amyloid fibril based on interstrand alignment of an antiparallel-sheet comprising a C-terminal peptide. Nat. Struct. Biol. 2, 990-998.
20. Han, H., Weinreb, P.H., and Lansbury, P.T.,Jr. (1995). The core Alzheimer’s peptide NAC forms amyloid fibrils which seed and are seeded by beta-amyloid: is NAC a common trigger or target in neurodegenerative disease? Chem. Biol. 2, 163-169.
21. Weinreb, P.H., Zhen, W., Poon, A.W., Conway, K.A., and Lansbury, P.T.,Jr. (1996). NACP, a protein implicated in Alzheimer’s disease and learning, is natively unfolded. Biochemistry 35, 13709- 13715.
22. Lee, S.J., Liyanage, U., Bickel, P.E., Xia, W., Lansbury, P.T.,Jr, and Kosik, K.S. (1998). A detergent-insoluble membrane compartment contains A beta in vivo. Nat. Med. 4, 730-734.
23. Evans, K.C., Berger, E.P., Cho, C.G., Weisgraber, K.H., and Lansbury, P.T.,Jr. (1995). Apolipoprotein E is a kinetic but not a thermodynamic inhibitor of amyloid formation: implications for the pathogenesis and treatment of Alzheimer disease. Proc. Natl. Acad. Sci. U. S. A. 92, 763-767.
24. Lashuel, H.A., Hartley, D.M., Petre, B.M., Wall, J.S., Simon, M.N., Walz, T., and Lansbury, P.T.,Jr. (2003). Mixtures of wild-type and a pathogenic (E22G) form of Abeta40 in vitro accumulate protofibrils, including amyloid pores. J. Mol. Biol. 332, 795-808.
25. Kheterpal, I., Lashuel, H.A., Hartley, D.M., Walz, T., Lansbury, P.T.,Jr, and Wetzel, R. (2003). Abeta protofibrils possess a stable core structure resistant to hydrogen exchange. Biochemistry 42, 14092-14098.
26. Spillantini, M.G., Crowther, R.A., Jakes, R., Hasegawa, M., and Goedert, M. (1998). alpha-Synuclein in filamentous inclusions of Lewy bodies from Parkinson’s disease and dementia with lewy bodies. Proc. Natl. Acad. Sci. U. S. A. 95, 6469-6473.
27. Spillantini, M.G., Schmidt, M.L., Lee, V.M., Trojanowski, J.Q., Jakes, R., and Goedert, M. (1997). Alpha-synuclein in Lewy bodies. Nature 388, 839-840.
28. Giasson, B.I., Uryu, K., Trojanowski, J.Q., and Lee, V.M. (1999). Mutant and wild type human alpha-synucleins assemble into elongated filaments with distinct morphologies in vitro. J. Biol. Chem. 274, 7619-7622.
29. Abeliovich, A., Schmitz, Y., Farinas, I., Choi-Lundberg, D., Ho, W.H., Castillo, P.E., Shinsky, N., Verdugo, J.M., Armanini, M., Ryan, A., et al. (2000). Mice lacking alpha-synuclein display functional deficits in the nigrostriatal dopamine system. Neuron 25, 239-252.
30. Conway, K.A., Harper, J.D., and Lansbury, P.T. (1998). Accelerated in vitro fibril formation by a mutant alpha-synuclein linked to early-onset Parkinson disease. Nat. Med. 4, 1318-1320.
31. Conway, K.A., Lee, S.J., Rochet, J.C., Ding, T.T., Williamson, R.E., and Lansbury, P.T.,Jr. (2000). Acceleration of oligomerization, not fibrillization, is a shared property of both alpha-synuclein mutations linked to early-onset Parkinson’s disease: implications for pathogenesis and therapy. Proc. Natl. Acad. Sci. U. S. A. 97, 571- 576.
32. Conway, K.A., Harper, J.D., and Lansbury, P.T.,Jr. (2000). Fibrils formed in vitro from alpha-synuclein and two mutant forms linked to Parkinson’s disease are typical amyloid. Biochemistry 39, 2552-2563.
33. Conway, K.A., Rochet, J.C., Bieganski, R.M., and Lansbury, P.T.,Jr. (2001). Kinetic stabilization of the alpha-synuclein protofibril by a dopamine-alpha-synuclein adduct. Science 294, 1346-1349.
34. Volles, M.J., and Lansbury, P.T.,Jr. (2007). Relationships between the sequence of alpha-synuclein and its membrane affinity, fibrillization propensity, and yeast toxicity. J. Mol. Biol. 366, 1510- 1522.
35. Vamvaca, K., Volles, M.J., and Lansbury, P.T.,Jr. (2009). The first N-terminal amino acids of alpha-synuclein are essential for alpha- helical structure formation in vitro and membrane binding in yeast. J. Mol. Biol. 389, 413-424.
36. Park, J.Y., and Lansbury, P.T.,Jr. (2003). Beta-synuclein inhibits formation of alpha-synuclein protofibrils: a possible therapeutic strategy against Parkinson’s disease. Biochemistry 42, 3696-3700.
37. Cuervo, A.M., Stefanis, L., Fredenburg, R., Lansbury, P.T., and Sulzer, D. (2004). Impaired degradation of mutant alpha-synuclein by chaperone-mediated autophagy. Science 305, 1292-1295.
38. Martinez-Vicente, M., Talloczy, Z., Kaushik, S., Massey, A.C., Mazzulli, J., Mosharov, E.V., Hodara, R., Fredenburg, R., Wu, D.C., Follenzi, A., et al. (2008). Dopamine-modified alpha-synuclein blocks chaperone-mediated autophagy. J. Clin. Invest. 118, 777-788.
39. Das, C., Hoang, Q.Q., Kreinbring, C.A., Luchansky, S.J., Meray, R.K., Ray, S.S., Lansbury, P.T., Ringe, D., and Petsko, G.A. (2006). Structural basis for conformational plasticity of the Parkinson’s disease-associated ubiquitin hydrolase UCH-L1. Proc. Natl. Acad. Sci. U. S. A. 103, 4675-4680.
40. Liu, Z., Meray, R.K., Grammatopoulos, T.N., Fredenburg, R.A., Cookson, M.R., Liu, Y., Logan, T., and Lansbury, P.T.,Jr. (2009). Membrane-associated farnesylated UCH-L1 promotes alpha- synuclein neurotoxicity and is a therapeutic target for Parkinson’s disease. Proc. Natl. Acad. Sci. U. S. A. 106, 4635-4640.
News Contact
[Role-playing Peter T. Lansbury, Center for Neurologic Diseases, Brigham and Women’s Hospital and Department of Neurology, Harvard Medical School, Cambridge MA 02142]This author wrote the paper for Biology 346: Molecular Neuroscience taught by Dr. Shubhik DebBurman.