The Remarkable Effects of Misfolded Proteins: Infection, Evolution, & Disease
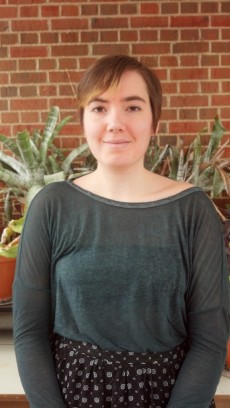
Katrina Campbell
Biology Department
Lake Forest College
Lake Forest, IL, 60045
Download PDF
The diversity of proteins and their extraordinary range of functions control the great diversity of life. In order for a protein to function correctly, it must fold properly. Many misfolded proteins have been linked to disease and death. My lab has sought to ask what organisms do to prevent misfolding and if misfolding is always detrimental. We and others discovered an ancient family of proteins called heat shock proteins (Hsps) that diversely assist in protein folding in all living organisms. Some Hsps even disaggregate misfolded proteins, preventing death. Stanley Prusiner first defined prions as alternately folded protein shapes that acted as infectious agents in mammals. Through our work with several yeast prions, we have expanded this definition to serve also as an agent of inheritance (a gene). In fact, we have demonstrated several yeast prions in the wild, where they exert evolutionary benefit in some environments. We also demonstrated that the mammalian prion protein is highly neurotoxic in the cytoplasm even when non-infectious and is important for maintaining stem cell pools in the brain that are necessary for neurogenesis. Finally, we have pioneered yeast models for many neurodegenerative diseases that have led to new pathogenic insights and novel therapy avenues. Our work has furthered the understanding of the fundamental nature of protein folding, what genes are, and uncovered the unexpected conclusion that misfolded proteins can drive evolution, just as they can cause disease.
Introduction
Proteins are the work horses of the cell. They act as messengers, provide structural support, transport molecules, and perform many other necessary functions. Proteins have been conserved throughout evolutionary history and are the most important regulators of cellular processes. Protein folding is central to protein activity. In the past, misfolded proteins have been linked to diseases that can be caused by the loss of a necessary function provided by the protein or by the new, toxic function of the misfolded protein.
Heat Shock Proteins
When a protein folds, it adopts the most thermodynamically stable shape. The protein just translated by a ribosome is unfolded. The protein moves stochastically, adapting many conformations. Stabilizing interactions are kept and the protein adapts its final shape through trial and error. This system works in vitro, but an unfolded protein in a cell filled with other macromolecules may experience other interactions, such as pH, that affect its folding. Even if the protein consists of the appropriate amino acids in the right order, if the proper shape is not achieved, the protein cannot continue normal function. A class of proteins called chaperone exist to assist proteins in finding the appropriate shape. These proteins are found all cell types and interact with proteins during different stages of folding. Chaperones do no assist in any step of the folding process, but protect the protein from the cellular environment to increase effectiveness of folding to reduce the chance of misfolding. During times of cellular stress such as elevated temperatures, chaperone proteins are particularly important. After exposure to high temperatures, all cells produce a type of chaperone protein known as heat shock proteins (Hsps). Once a cell has been exposed to an elevated temperature and heat shock proteins have been produced, the cell is more viable in extreme temperatures than cells that have not been exposed to a moderately elevated temperature.
Hsp104 is highly conserved
Hsp104 is found in yeast cells and is essential for stress tolerance. Sequence analysis of Hsp104 revealed two nucleotide-binding sites. Hsp104 is very similar to the ClpA/ClpB family of proteins. The two nucleotide binding sites of Hsp104, ClpA, and ClpB are all homologous. The homology between Hsp104 and ClpB extends past the binding sites. Similarly to Hsp104, ClpB is also induced at elevated temperatures and reacts to Hsp104 antibody. From this data, it was concluded that Hsp104 is a member of the ClpB family. The mammalian heat shock protein Hsp110 also reacts to the Hsp104 antibody, indicating that the yeast Hsp104 and mammalian Hsp110 are homologs, and are thus conserved. The ability of the two nucleotide binding sites of Hsp104 to bind ATP is essential to protect against extreme heat. This loss in protection is not due to a structural or mobility change in Hsp104. Hsp104 is a significant protein that has been conserved through time.
Hsp104 confers general stress tolerance
In addition to cells exposed to high temperatures, Hsps are also induced by a large variety of other stressors such as ethanol and metals. Cells pretreated to a mild heat show an increased tolerance to high heat when compared to cells without the treatment. These pretreated cells also show an increased tolerance to ethanol than other cells. Yeast cells with mutated, nonfunctional Hsp104 grow at the same rate as wild type cells at low temperatures. These cells also die at the same rate when exposed directly to high heats without pretreatment. When the wild type yeast cells are pretreated, they show increased thermotolerance to high heat. The cells with the mutated Hsp104; however, show only very transient thermotolerance and quickly die. This indicates that Hsp104 is required for acquisition of thermotolerance. When yeast cells with functional Hsp104 are pretreated with ethanol, cells not only develop an increased tolerance to ethanol, but also to heat. This tolerance does not develop if the cells do not possess functional Hsp104. Similar experiments were done on sodium arsenite suggest that Hsp104 is also part of the stress response to this stimuli. Hsp104 does not give the same tolerance to the tested metals, copper and cadmium.
When yeast cells are grown on glucose, cells mainly grow by fermentation, repressing respiration, and produce ethanol as a by-product. When cells are grown in acetate media, they grow by respiration as opposed to fermentation. Cells growing by respiration produce less dense colonies than those growing by fermentation. Yeast cells that undergo respiration also produce heat shock proteins and have an Hsp104-dependent tolerance to heat exposure. This decrease in cell density but increase in Hsp production is likely an evolutionary tradeoff. Yeast cells ‘prefer’ to grow by fermentation if glucose is present. This produces ethanol. Once the glucose reservoirs have been depleted and the ethanol levels are high, the cells switch to using respiration. The production of Hsp104 likely helps the cells survive the high concentrations of ethanol. Hsp104 provides tolerance, not just to heat, but to a myriad of other forms of stress.
Hsp104 disaggregates proteins
The Clp family to which Hsp belongs is a family of proteases. Hsp104; however, does not seem to be a protease itself. Yeast cells with either normal or mutant, nonfunctional, Hsp104 were pretreated with a mild heat, and then exposed to a high heat. Luciferase is an enzyme that aggregates in cells exposed to high levels of heat. Protein synthesis was blocked after exposure. In cells with nonfunctional Hsp104, luciferase did not regain enzymatic activity after the treatment. In cells with functional Hsp104, luciferase activity was regained after treatment. Hsp104 resolubilizes luciferase after aggregation during treatment. The functional ability of the two nucleotide binding sites is imperative for the ability of Hsp104 to mediate luciferase function rescue. This rescue ability seems limited to Hsp104 and not Hsps in general. Hsp 104 mediates thermotolerance through its ability to disaggregate proteins aggregated by stress.
Yeast Prions
The central dogma in protein genetics is the idea that proteins are made in one way. DNA is transcribed into RNA and this RNA is translated into a protein. Prions challenge this concept. Prions are proteins that can act as genes (see figure 1). These proteins adopt alternative shapes and act as a template to convert traditionally folded protein to the alternative form.
The [psi+] factor in yeast closely resembles a prion. [psi+] is inherited in a non-Mendelian fashion and has not found to be coded for by DNA or RNA. Sup35 is the basis for the [psi+] factor in yeast. Sup35 is a translation release factor. When in the [psi-] form, Sup35 recognizes the termination code in RNA during translation and stops protein synthesis at the appropriate time. When in the [psi+] form; however, nonsense mutations are common.
To serve as controls, lab strains of yeast often carry mutations of essential genes that normally allow them to grow on deficient media. These genes carry a transcription termination code in the middle of the gene so under normal conditions, the protein is
truncated and is nonfunctional. The plasmids inserted into these cells carry the essential genes, allowing the cell to survive on deficient media. It was noted that some laboratory strains of Saccharomyces cerevisiae could grow on defiant media without a plasmid insertion. This indicates that a nonsense mutation has taken place and the yeast is somehow still manufacturing a full version of the mutated protein. Since Sup35 is a translation release factor, and mutations of Sup35 result in nonsense mutations, it is likely that instead of truncating the proteins at the desired spot, read-through occurs and the ribosome continues to manufacture a full, functional protein.
Figure 1: Prions can act as agents of infection or inheritance. Mammalian prions can act as agents of infection and cause disease. Yeast prions can act as agents of inheritance and cause non-Mendelian inheritance of the prion state.
Role of Hsp104 in [psi+] propagation
[psi+] can be lost from a cell after stress. Screening identified the gene encoding Hsp104 as an inhibitor of [psi+]-mediated termination suppression. Transiently expressing Hsp104 suppressed [psi+], but did not eliminate it as [psi+] expression was recovered once Hsp104 expression was suppressed. Interestingly, in cells with mutant Hsp104 plasmid with nonfunctional binding sites, the [psi+] state was ‘cured’ and the cells permanently became [psi-]. In Hsp104Δ strains, the [psi+] state was also lost. Addition of multiple Sup35 genes has been shown to promote conversion from the [psi+] to
the [psi+] state. In [psi-] Hsp104+ cells, multicopy Sup35 caused an increase in the conversion to the [psi+] state. In Hsp104Δ cells; however, the cells did not convert to the [psi+] state. Both a large and a small amount of Hsp104 result in [psi+] state suppression. Thus, an intermediate amount of Hsp104 is necessary for [psi+] state propagation. Hsp104 is a chaperone protein that disaggregates proteins. This suggests that Hsp104 disaggregates Sup35, allowing it to form its prion shape.
The N terminal and the M domain of Sup35 are necessary and sufficient for maintenance of the [psi+] state and the transition between the [psi+] and [psi-] states. A protein consisting of the N and M domains of Sup35 will be referred to as NM throughout the rest of this paper. In [psi+] cells, NM is in an amyloid-like conformationand forms amyloid fibers in vitro. Exposing [psi-] S. cerevisiae cells to NM amyloid fibers induces the [psi+] state, like a true prion. Addition of Hsp104 and ATP to NM greatly increases the rate at which NM forms amyloid fibers. Generally, when NM forms fibers, a lag time exists during which there is very little growth. The addition of Hsp104 in conjunction with ATP drastically shortened this lag time. The NM fibers formed through Hsp104 interaction are very similar to those formed in spontaneous reactions and can act as seeds to catalyze the polymerization of soluble NM. Importantly, NM fibers formed from HSP104 interaction can cause a state change from [psi-] to [psi+]. Before NM form amyloid fibers, it exists in an oligomeric state. Sequestration of these oligomers prevents the formation of fibers. The oligomeric species; however, is not necessary for the propagation of fibers once they have formed. Conversely, the presence of fibers is crucial for the fibrilization of both seeded and unseeded reactions. Hsp104 eliminates the lag time of fiber formation because it catalyzes the formation of NM oligomers. ATP is necessary for the Hsp104-driven catalysis of NM fibrilization. Mutant Hsp104 with inactive ATP binding sites or Hsp104 with ADP is ineffective in promoting fibrilization. Hsp104 also breaks up NM fibers, exposing novel surfaces for soluble NM to build off. When Hsp104 with ATP was present at high concentrations, NM fibrilization was inhibited. Hsp104 eliminated NM oligomer formation, blocking fiber formation. Without ATP, Hsp104 did not degrade oligomers, but prevented them from becoming fibers. At low concentrations, Hsp104 promotes NM fiber formation, but at high concentrations, Hsp104 inhibits fiber formation. This is reflective of [psi-] state formation and propagation with low or high levels of Hsp104 expression.
The molecular basis of the [psi-] state
When Sup35 is expressed at very high levels in cells, Hsp104 is not required for the maintenance of the [psi-] state. The N region of Sup35 is the only region that incites the [psi-] state in Escherichia coli. The M region does not induce the [psi-] state and the C region of Sup35 works to counteract the [psi-] state. Both the M and C regions accumulate as soluble proteins, even when at high levels. Congo red binding patterns indicate that fibers formed by the whole Sup35 protein and NM are amyloid fibers. NM fiber formation is greatly influenced by concentration of NM and experiences a large lag time. This pattern of formation indicates that the rate limiting step in fibril formation is nucleation. The addition of a small amount of seed fibers to soluble NM eliminated the lag time and supports this theory. Lysates from [psi+] cells also eliminate the lag time. Together, these data support the hypothesis that [psi+] is a prion state.
There are many similarities between yeast prion Sup35 and mammalian prion PrP. Both include oligopeptide-repeat expansions. These expansions in the Sup35 protein reside in [psi+]-mediating N terminus. The Repetition of these expansions in the PrP protein results in spontaneous prion formation and propagation. The oligopeptide repeats found in Sup35 in S. cerevisiae are crucial for the maintenance of the [psi+] state. Similarly to the PrP protein, repeats of the oligopeptide expansions increases the rate of spontaneous [psi+] elements. Also, with repeat expansions, the rate at which Sup35 forms β sheets and fibers is increased. The oligopeptide repeats found in Sup35 mediate the inheritance pattern of the [psi+] state.
In the [psi+] state, the N and M regions of Sup35 change conformation. These conformation changes are replicated and convert soluble Sup35 to the same conformation. The ends, as opposed to the lateral sides, of NM serve as the template for further NM binding. NM amyloid formation is perpetuated through a model called the nucleated conformational conversion (NCC). In this model, NM fibers serve as a template. NM fibers are formed by unstructured oligomeric intermediates. When only intermediate oligomers exist, a lag time reflects the time it takes for the first fiber to form. Once a template is formed, polymerization continues rapidly.
Yeast prions can serve as genes and provide phenotypic variation
[psi+] and [psi-] cells from different S. cerevisiae strains produce a large variation in phenotype when characterized using a large number of phenotypic assays. In about half of the assays, the [psi] state of the cell made a significant difference in the growth rate of the cell. In over twenty-five percent of these tests, the [psi+] state conferred an advantage. The assays produced a myriad of results. In some strains, there was no difference between [psi] states, whereas for the same assay, other strains would show a difference. Also, while the [psi+] state conferred an advantage to some cells in a test, another strain might be disadvantaged by the [psi+] state. The [psi] state of the cell also caused a difference in the visual appearance of colonies. Through the [psi+] state, otherwise hidden genetic variation within yeast cells are expressed and cause phenotypic variation. The differences in phenotype are linked directly to the nonsense suppression caused by the [psi+] state.
The ability of yeast to switch spontaneously between the [psi] states is likely evolutionarily significant. In a large population of S. cerevisiae, both [psi-] and [psi+] states are likely expressed in some cells. If the environment is more suitable for [psi-] cells, for example, these cells will persist, while the [psi+] cells may die or have decreased survival. The [psi+] state; however, is not lost from the population. A small number of [psi-] cells will switch spontaneously to the [psi+] state under normal conditions. If the environment changes to be more suited for [psi+] cells, some will likely exist in the population and thrive.
Support for the prion hypothesis in yeast prions
The NM region of Sup35 is the prion-inducing region. The NM region was attached to a glucocorticoid receptor protein. This recombinant protein acted as a prion. Overexpression caused a heritable state change that was inherited in a protein-only manner.
Sup35 is insoluble in [psi+] cells. Sup35 is reverted back to its solid state by overexpression of functional Hsp104. The [psi+]-mediated nonsense suppression is due to the conformational change in Sup35. Furthermore, Sup35, when over expressed in E. coli cells, attains the prion conformation. The prions formed in E. coli cells are infectious. Together, these data confirm that Sup35 is the basis for the [psi] state in S. cerevisiae and confirm the prion hypothesis for Sup35 in yeast.
Prions exist outside of Sup35 in laboratory yeast strains
A bioinformatics screen was used to test the possibility that undiscovered prions exist in S. cerevisiae. About 200 proteins in the S. cerevisiae genome have domains similar to prion domains. Out of 29 proteins identified to be prion candidates, [MOT+] was thoroughly investigated and found to be a novel prion.
If benign or even beneficial prions, as has been described thus far, only exist in laboratory strains of S. cerevisiae, the implications of yeast prion research is slim. It has been found; however, that prions are a common way in which phenotypes are inherited in wild yeasts. The Sup35 prion exists, not only in laboratory strains of yeast, but in wild yeasts as well. [psi] states act similarly in the wild strains as they do it the laboratory strains, and expresses natural variation. [RNQ+] also exists both in laboratory and wild strains of yeasts. Wild strains also have additional prions that had not been identified in a lab setting. Prions are common in all strains of yeast tested, laboratory and wild, implying a biological significance of prions to yeast.
The cytoplasmic polyadenylation element binding protein (CPEB) in the Aplysia caliornica shares many features with yeast prions. The N terminal domain shares qualities with the prion-determining domain of other prion proteins. An N terminal-GFP fusion protein produces two distinct, heritable states. These states are inherited in a non-Mendelian fashion. The full CPEB protein also exists in two distinct, heritable states. Interestingly, the prion-like state of CPEB can bind RNA, and is actually the more active form of the protein38. The discovery of a non-pathogenic prion-like protein in a neuron of an animal has many implications for prion research in the future. This opens the possibility that not all prions in animals are pathogenic and some may be beneficial, like yeast prions.
The effects of chaperones on yeast prions
After stress, Hsp104 works in conjunction with Hsp40 and Hsp70 to disaggregate and refold proteins. They also impact the Hsp104-mediated propagation or suppression of the [psi+] state in S. cerevisiae.
Chaperones have been shown to affect prion states in yeasts. [RNQ+] is an identified yeast prion. Chaperone proteins are necessary for the maintenance of the [RNQ+] state. Hsp40 prevents toxicity caused by overexpression of Rnq1, but does so by promoting the [RNQ+] state instead of the soluble, [RNQ-] state. This suggests that without chaperone direction, the Rnq1 protein forms toxic conformers. When Rnq1 is sequestered, these conformers do not form and thus do not become toxic.
Figure 2: α-synuclein inhibits ER-Golgi trafficking. α-synuclein increased ER stress and inhibits ER to Golgi trafficking in S. cerevisiae cells.
Mammalian Prions
In mammals, transmissible spongiform encephalopathies (TSEs), are linked to the mammalian prion, PrP. PrP is crucial for disease spread(seefigure 1). When in the prion form, PrP is
written PrPsc.
PrP is expressed in the cytosol of living cells
In order to confirm that PrP is indeed a prion, it must be able to be expressed in a non-mammalian cell and still be infectious.
It is known that PrP is expressed on the cellular membrane of cells, but when PrP is expressed in S. cerevisiae cells, some of the PrP appears to be expressed in the cytoplasm43. This protein is soluble and proteinase K resistant. This protein, however, is not PrPsc and is not infectious or toxic like PrPsc. When proteasome activity is impaired, insoluble PrP accumulates in the cytosol of yeast cells. This PrP was not broken down once proteasome activity was restored, indicating that it is PrPsc-like. When Prp is expressed in the cytosol of cells, it is toxic. Mice with neuronal cytosolic PrP exhibited motor deficiencies and neurotoxicity.
The role of PrP
Despite the fact that it has been the subject of many studies, the role of PrP in a healthy brain has not received very much attention. PrP up-regulates the amount of neural proliferation in adult mice. PrP is also important for the regeneration of hematopoietic stem cells.
Proof that PrP is a prion
To prove that TSEs are not caused by a low-acting virus, PrP protein was recombinantly expressed in E. coli. cells. This PrP protein aggregated, became protease-resistant, and was self-perpetuating. Importantly, when this protein was transferred from the bacteria into wild-type mice, the mice developed a prion disease. This infectivity and toxicity confirm that PrP is a prion.
Parkinson’s Disease (PD)
Yeast as a model for PD
Parkinson’s Disease, a synucleinopathy, is a protein misfolding disorderlinked to the misfolding of α-synuclein. Because yeasts make, fold, and degrade proteins just like humans do, they are a viable candidate to model α-synuclein. When α-synuclein is expressed in a S. cerevisiae, it enhances lipid accumulation, affects vesicle pools and binds to membranes. Genome-wide screens were done on yeast with Huntingtin or α-synuclein added. The screens identified genes that enhance the toxicity of either Huntingtin or α-synuclein. The genes that tended to increase the toxicity of Huntingtin are the gene families responsible for stress response, protein folding, and Ubiquitin-dependent catabolism. The genes that mediate α-synuclein toxicity tended to be genes concerning lipid metabolism and vesicle transport. α-synuclein inhibits ER-Golgi trafficking (see figure 2). This trafficking deficiency is rescued by the overexpression of Ypt1p, a protein that promotes trafficking. α-synuclein induced neuron loss is mitigated by Rab1, a Ypt1p ortholog.
Possible Parkinson’s Disease therapy pathways
Novel therapy pathways are crucial for PD, a disease which has no cure and no effective long-term therapy. Ways to ameliorate the toxicity of α-synuclein also gives insights as to how α-synuclein creates toxicity in the first place. Chemicals were found that rescue ER-Golgi trafficking defects and mitochondrial function that were destroyed by α-synuclein. Cyclic peptides that reduce α-synuclein toxicity in S. cerevisiae and C. elegans were also found. These cyclic peptides work downstream of the vesicle defects caused by α-synuclein.
Conclusion
Together, the different topics I have worked on further our knowledge of how proteins interact, how proteins can act as genes or infectious agents, and how we can use model organisms to model diseases in humans.
Acknowledgements:
I would like to thank Dr. DebBurman for his assistance and advice throughout the research phase of this paper. I would also like to thank all my Bio346 classmates for their support.
References:
Vendruscolo, M., Zurdo, J., MacPhee C.E., & Dobson, C.M. (2003). Protein folding and misfolding: a paradigm of self-assembly and regulation in complex biological systems. Phil. Trans. R. Soc. Lond. 361:1205-1222.
Winklhofer, K.F., Tatzelt, J., & Haass C. (2008). The two faces of protein misfolding: gain- and loss-of-function in neurodegenerative diseases. The EMBO journal, 27(2):336-349.
Dobson, C.M., SÏ ali, A., & Karplus, M. (1998). Protein folding: a perspective from theory and experiment. Angew. Chem. Int. Ed., 37:868-893.
Wolynes, P.G, Onuchic, J.N., & Thirumalai, O.D.(1995). Navigating the folding routes. Science, 267:1619-1620.
Dobson, C.M. (2003). Protein folding and misfolding. Nature, 426:884-890.
Ellis R.J. (2001). Macromolecular crowding: an important but neglected aspect of the intracellular environment. Curr. Opin. Struct. Biol., 11(1):114-119.
Ellis, R.J., & van der Veis, S.M. (1991). Molecular chaperones. Annu. Rev. Biochem., 60:321-347.
Lindquist, S., & Craig, E.A., (1988). The heat-shock proteins. Annu. Rev. Genet., 22:631-677.
Sanchez, Y., Taulein, J., Borkovich, K.A., Lindquist, S. (1992). Hsp104 is required for tolerance to many forms of stress. The EMBO jour., 11(6):2357-2364.
Gotresman, S., Squires, C., Picheresky, E., Carringtion, M., Hobbsii, M., Mattickii, J.S., Dalrymple, B., et al. (1990). Conservation of the regulatory subunit for the Clp ATP-dependent protease in prokaryotes and eukaryotes. Proc. Natn. Acad. Sci. U.S.A., 87:3515-3517.
Parsell, D.A., Sanchez, Y., Stitzel, J.D. & Lindquist, S. (1991). Hsp104 is highly conserved with two essential nucleotide-binding sites. Nature, 353: 270-273.
Plesset, J., Palm, C. & McLaughlin, C.S. (1982). Induction of heat shock proteins and thermotolerance by ethanol. Biochem. Biophys. Res. Commun., 108(3):1340-1345
Käppeli, O. (1987). Regulation of carbon metabolism in Saccharomyces cerevisiae and related yeasts. Adv. Microb. Physiol., 28: 181-209.
Katayama-Fujimura, Y, Gottesman, S. & Maurizi, M.R. (1987). A multiple-component, ATP-dependent protease from Escherichia coli. J. Biol. Chem., 262:4477-4485.
Parsell, D.A., Kowal, A.S., Singer, M.A. & Lindquist, S. (1994). Protein disaggregation mediated by heat-shock protein Hsp104. Nature, 372: 475-478.
Wang, K. & Kurganov, B.I. Kinetics of heat- and acidification-induced aggregation of firefly luciferase. Biophys. Chem., 106(2):97-109.
Cox, B. (1994). Cytoplasmic inheritance: prion-like factors in yeast. Curr. Biol., 4(8): 744-748.
Cox, B.S., Tuite, M.F. &McLaughlin, C.S. (1988). The Ψ factor of yeast: a problem in inheritance. Yeast, 4: 159-178.
Stansfield, I., Jones, K.M., Kushnirov, V.V., Dagkesamanskava, A.R, Poznyakovski, A.I., Paushkin, S.V., Nierras, C.R., Cox, B.S., Ter-Avanesyan, M.D. & Tuite, M.F. (1995). The products of the SUP45 (eRF1) and SUP35 genes interact to mediate translation termination in Saccharomyces cerevisiae. EMBO J., 14(17):4365-4373.
Chernoff, Y.O., Lindquist, S.L., Ono, B.-I., Inge-Vechtomov, S.G. & Leibman, S.W. (1995). Role of the chaperone protein Hsp104 in propagation of the yeast prion-like factor [psi+]. Science, 268:880-883.
Chernoff, Y.O., Derkach, I.L. & Inge-Vechtomov, S.G. (1993). Multicopy SUP35 gene induces de-novo appearance of psi-like factors in the yeast Saccharomyces cerevisiae. Curr. Genet. 24(3):268-270.
Patino, M.M., Liu, J.-J., Glover, J.R. & Lindquist, S. (1996). Support for the prion hypothesis for inheritance of a phenotypic trait in yeast. Science, 273(5275):622-626.
Tanaka, M., Chien, P., Naber, N., Cooke, R. & Weissman, J. (2004). Conformational variations in an infectious protein determine prion strain differences. Nature, 428:323-328.
Shorter, J. & Lindquist, S. (2004). Hsp104 catalyzes formation and elimination of self-replicating Sup35 prion conformers. Science, 304: 1793-1797.
Glover, J.R., Kowal, A.S., Schirmer, E.C., Patino, M.M., Liu, J.-J. & Lindquist, S. (1997). Self-seeded fibers formed by Sup35, the protein determinant of [PSI+], a heritable prion-like factor of Saccharomyces cerevisiae. Cell, 89:811-819.
Kretzschmar, H.A., Stowring, L.E., Westaway, D., Stubblebine, W.H., Prusiner, S.B. & Dearmond, S.J. (2009). Molecular cloning of a human prion protein cDNA. DNA, 5(4):315-324.
Kushnirov, V.V., Ter-Avanesyan, M.D., Telckov, M..V., Surguchov, A.P., Smirnov, V.N. & Inge-Vechtomov, S.G. (1988). Nucleotide sequence of the SUP2 (SUP35) gene of Saccharomyces cerevisiae. Gene, 66(1): 45-54.
Prusiner, S.B. & Scott, M.R. (1997). Genetics of Prions. Annu. Rev. Genet., 31: 139-175.
Liu, J.-J. & Lindquist, S. (199). Oligopeptide-repeat expansions modulate ‘protein only’ inheritance in yeast. Nature, 400: 573-576.
Serio, T.R., Cashikar, A.G., Kowal, A.S., Sawicki, G.J., Moslehi, J.J., Serpell, L., Arnsdorf, M.F. & Lindquist, S. (2000). Nucleated conformational conversion and the replication of conformational information by a prion determinant. Science, 289: 1317-21.
True, H.L., Lindquist, S.L. (2000). A yeast prion provides a mechanism for genetic variation and phenotypic diversity. Nature, 431: 184-187.
True, H.L. & Lindquist, S.L. (2004). Epigenetic regulation of translation reveals hidden genetic variation to produce complex traits. Nature, 407: 477-483.
Lund, P.M. & Cox, B.S. (1981). Reversion analysis of [psi-] mutations in Saccharomyces cerevisiae. Genet. Res., 37(2): 173-182.
Li, L. & Lindquist, S, (2000). Creating a protein-based element of inheritance. Science, 287: 661-664.
Garrity, S.J., Slvanathan, V., Dong, J., Lindquist, S. & Hochschild, A. (2010). Conversion of a yeast prion protein to an infectious form in bacteria. PNAS, 107(23):10596-10601.
Alberti, S., Halfmann, R., King, O., Kapila, A. & Lindquist, S. (2009). A systematic survey identifies prions and illuminates sequence features of prionogenic proteins. Cell, 137:146-158.
Halfmann, R., Jarosz, D.F., Jones, S.K., Chang, A., Lancaster, A.K. & Lindquist, S. (2012). Prions are a common mechanism for phenotypic inheritance in wild yeasts. Nature, 482(7385):363-368.
Si, K., Lindquist, S. & Kandel, E.R. (2003). A neuronal isoform of the aplysia CPEB has prion-like properties. Cell, 115:879-91.
Glover, J.R., Kowal, A.S., Schirmer, E.C., Patino, M.M, Liu, J.J. & Lindquist, S. (1977). Self-seeded fibers formed by Sup35, the protein determinant of [PSI+], a heritable prion-like factor of S. cerevisiae. Cell, 89: 811-819.
Shorter, J. & Lindquist, S. (2008). Hsp104, Hsp70 and Hsp40 interplay regulates formation, growth and elimination of Sup35 prions. EMBO J, 27: 2712-2724.
Douglas, P.M., Treusch, S., Ren, H.Y., Halfmann, R., Duennwald, M.L., Lindquist, S. & Cyr, D.M. (2008). Chaperone-dependent amyloid assembly protects cells from prion toxicity. PNAS, 105(20):7206-11.
Prusiner, S.B. (1998). Prions. Proc. Natnl. Acad. Scien. U.S.A., 95(23):13363-13383.
Ma, J. & Lindquist, S. (1999). De novo generation of PrPSc-like conformation in living cells. Nat Cell Biol, 1(6): 358-361.
Ma, J. & Lindquist, S. (2002). Conversion of PrP to a self-perpetuating PrPSc-like conformation in the cytosol. Science, 298(5599):1785-17888.
Ma, J., Wollman, R. & Lindquist, S. (2002). Neurotoxocity and neurodegeneration when PrP accumulates in the cytosol. Science, 298: 1781-1785.
Steele, A.D., Emsley, J.G., Özdinler, P.H., Lindquist, S & Macklis, J.D. (2006). Prion protein (PrPc) positively regulates neural precursor proliferation during developmental and adult mammalian neurogenesis. Proc. Nat. Acad. Sci., 103(9):3416-3421.
Zhang, C.C., Steele, A.D., Lindquist, S. & Lodish, H.F. (2005). Prion protein is expressed on long-term repopulating hematopoietic stem cells and is important for their self-renewal. Proc. Nat. Acad. Sci., 103(7): 2184-2189.
Wang, F., Wang, X., Yuan, C.-G. & Ma, J. (2010). Generating a prion with bacterially expressed recombinant prion protein. Science, 327(5969):1132-1135.
Muchowski, P.J. (2002). Protein misfolding, amyloid formation, and neurodegeneration. Neuron, 35(1): 9-12.
Willingham, S., Fleming, Outeiro, T., DeVit, M.J., Lindquist, S.L. & Muchowski, P.J. (2003). Yeast genes that enhance the toxicity of a mutant Huntingtin fragment or α- synuclein. Science, 302: 1769-1772.
Cooper, A.A., Gitler, A.D., Cashikar, A., Haynes, C.M., Hill, K.J., Bhullar, B., et al. (2006). Alpha-synuclein blocks ER-golgi traffic and rab1 rescues neuron loss in Parkinson’s models. Science, 313: 324-8.
Su, L.J., Auluck, P.K., Outeiro, T.F., Yeger-Lotem, E., Kritzer, J.A., Tardiff, D.F., et al. (2010). Compounds from an unbiased chemical screen reverse both ER-to-Golgi trafficking defects and mitochondrial dysfunction in Parkinson’s disease models. Dis Model Mech., 3:194-208.
Kritzer, J.A., Hamamichi, S., McCaffery, J.M., Santagata, S., Naumann, T.A., Caldwell, et al. (2009). Rapid selection of cyclic peptides that reduce alpha-synuclein toxicity in yeast and animal models. Nat. Chem. Biol., 5: 655-63.