FRAGILE X: Mental Retardation Caused by Expanded CGC and Silenced FMR
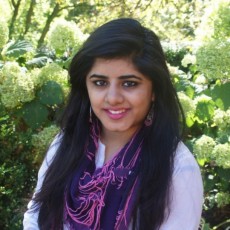
Rida Khan
Department of Neuroscience
Lake Forest College
Lake Forest, Illinois 60045
Download PDF
*This author wrote the paper as a part of BIOL346: Molecular Neuroscience under the direction of Dr. DebBurman.
Introduction
Clinical diagnoses of inherited mental retardation and autism encompass heterogeneous subsets of patients with differing molecular mechanisms of disease. For simplification of molecular analysis, the study of different forms of these broader diseases makes it possible to identify a homogeneous group of patients. The molecular and genetic knowledge of fragile X syndrome (FXS) has proven valuable in identifying the underlying molecular pathways of inherited mental retardation (Santoro, Bray, & Warren, 2012).
Fragile X syndrome (FXS) is the most common inherited cause of mental retardation with approximately 1 in 4000 males affected (Garber, Visootsak, & Warren, 2008). Behaviorally, FXS individuals have mild to severe intellectual disability, often with autism-like behavior. Other neurological symptoms include developmental delay and increased susceptibility to seizures. Neurons of FXS patients are found to have dense, immature dendritic spines. Physically, male FXS individuals show macroorchidism, which develops just before puberty. More subtle physical symptoms might include a long, narrow face with prominent ears, joint laxity and flat feet (Bray et al., 2012).
Further analysis of FXS pedigrees showed that FXS does not follow a typical inheritance pattern for an X-linked disease. Some pedigrees contained male obligate carriers with no symptoms of FXS. Furthermore, grandchildren of healthy male carriers developed FXS at much higher rates than did the siblings of the carriers. Therefore the penetrance for this disease increases in succeeding generations of a pedigree. This example of genetic assumption was called the Sherman Paradox (Bray et al., 2012).
There is currently no cure or successful treatment options for FXS, however, there are some medications for certain symptoms. Scientists need to develop therapies and ultimately a cure for the treatment of FXS.
It wasn’t until the turn of the twentieth century that scientist discovered that mental retardation has a sex-linked component. In 1890s, scientists found out there was an excess of males with mental retardation than females in prisons an in the general population. Initially, this high prevalence in males was thought to be due to ascertainment bias. In 1974, Lehrke argued that genes affecting intelligence are located in the X chromosome. In 1969, Lubs noticed an unusual chromosomal gap on the long arm of the X chromosome in mentally retarded males. In 1983, the location of this variant was determined to be Xq27.3 and became known as a fragile X chromosome (O’Donnell & Warren, 2002) due to their propensity to break under certain conditions (Bray et al., 2012).
After the discovery of the fragile sites in 1985, the molecular mechanism by which such sites exhibit fragility was unknown. Our lab has attempted to determine the molecular mechanism behind FXS through studying the gene responsible for FXS, FMR1. As seen in Figure 1, our lab has shown the role of FMR1 and its protein product FMRP in FXS pathology.
Figure 1. Pathophysiology of normal vs. Fragile X Syndrome neuron: Pathology of Fragile X Syndrome causes increased glutamate receptor activity, increased internalization of AMPA receptors, increased dendritic spine density, and aberrantly rigid microtubules as a result of de- creased FMRP expression due to FMR1 methylation-induced silencing.
FMR1
Localization of the disease locus
Before we could clone the gene responsible for FXS, we needed to perform genetic and physical mapping analysis to reduce the interval of interest on the X chromosome and strengthen the localization of the disease locus to the fragile site. In 1987 and 1990, we devised a method using somatic cell hybrid lines to mark the fragile site itself by selecting for X chromosome breakage and translocation when fragility was induced (Warren, Zhang, Licameli, & Peters, 1987; Warren et al., 1990). Somatic cell hybrids were produced from fusions of Chinese hamster ovary cells deficient in both hypoxanthinephosphoribosyltransferase (HRPT) and glucose-6-phosphate dehydrogenase (G6PD) with lymphoblasts from a male patient with fragile X syndrome. Since HRPT and G6PD are syntenic, flanking the fragile X site and HRPT+, G6PD- selection identified hybrid cells that segregate these markers. Such segregation, after induction of fragile X site, was shown to result from chromosomal breakage at or near the fragile site (Warren et al., 1987).A selective strategy was devised to recover the human chromosomal band Xq28 within somatic cell hybrids through fragile-X-site breakage. Availability of these somatic cell hybrids accelerated the high-resolution genomic analysis of Xq28 for the identification of the candidate gene for FXS loci mapping to this region (Warren et al., 1990).
Identification of the FMR1 gene
Within the region that flanks the fragile X-locus, a fragile X-related CpG-island was identified that was aberrantly hypermethylated in patients and most carriers (Vincent et al., 1991; Bell et al., 1991). The presence of the CpG island, implied the presence of a gene that is probably inactivated due to hypermethylation within a genomic region that includes the fragile X-associated hybrid breakpoints. The gene for FXS was cloned in 1991 and named fragile X mental retardation gene 1 (FMR1). FMR1 was associated with the CpG island and majority of the hybrid translocation breakpoints within a 5.1 kb fragment, contained both the CpG island and sequences at the 5’ end of FMR1. The association of the fragile X site with FMR1 was suggested since length variations were observed in patients only. A highly unusual trinucleotide repeat CGG was also found along with high expression of FMR1 in human brain were compelling evidence that FMR1 is important in the phenotype of inheriting the fragile X locus (Verkerk et al., 1991).
Characterization of FMR1
The hypermethylation of the CpG island indicated a possible regulatory mechanism of the mutation in FMR1 in FXS individuals. We were therefore interested in determining the levels of FMR1 mRNA in FXS patients, carriers and normal individuals. Significantly reduced levels of FMR1 mRNA were observed in the majority of male FXS patients. A correlation was observed between total methylation within the CpG island, in the fragment containing the fragile site, and absence of FMR1 . We also showed variation of the length of DNA at the fragile site in FXS patients (Kremer et al., 1991).
Two general classes of mutations were seen in FXS families. Premutations, where small inserts (100-600 bp) do not associate with phenotype and large inserts (600-4000 bp) in affected males. We determined the number of CGG repeats in genomic DNA from normal and FXS individuals to study length variation at the fragile X mutation site (Fu et al., 1991). The number of CGG repeat length was higher in Fragile X patients than normal individuals. As the allele size of premutations increased, the risk of the expansion to full mutation increased (Fu et al., 1991). It was still unclear whether the abnormal methylation occurred as a consequence of the expansion of the CGG-repeat and if this methylation was responsible for the transcriptional inactivation of the FMR1 gene in most affected males. Therefore we compared chorionic villi with fetal DNA of carrier mothers since their genetic background is similar and they represent identical mutation. FMR1 transcription was present in unmethylated chorionic villi despite an expanded full mutation suggested lack of transcription in fetal DNA is a consequence of DNA methylation and that transcription is able to proceed through CGG repeats (Sutcliffe et al., 1992).
Normal gene expression patterns of FMR1 showed its localization in regions of the brain and tubules of the testes, areas most implicated in FXS. The expression levels of FMR1 were high in early mouse embryos and decreased along development.
FMR1 protein product: FMRP
The normal function of the FMR1 protein (FMRP) and the mechanism by which its absence leads to mental retardation were key questions to understand FXS. Analyses of FMRP revealed highly conserved KH domain and an RGG box. The KH domain constitutes the RNP family involved in RNA-protein interactions. Therefore we further looked at the RNA binding properties of FMRP. FMRP exhibited specificity for RNA species that it interacted with. RNase treatment abolished FMRP capture as well. Increasing the levels of RNA showed increased the levels of bound FMRP, thus concluding that FMRP is an RNA binding protein (Ashley, Wilkinson, Reines, & Warren, 1993).
To elucidate the mechanism of FMRP absence in FXS, translation levels were analyzed. No change in mRNA level was observed when CGG repeats increased. However, as the number of repeats increased, FMRP levels decreased. This implied that lengthy repeats may impede translation. Translational suppression was therefore implicated in FXS due to CGG repeat expansion at FMR1. The 40s ribosome subunit was stalled and therefore translation initiation was shown to be specifically disabled due to expanded CGG repeats (Yue, Lakkis, Eberhart, & Warren, 1995).
The cellular localization of FMRP was observed to be in high expression in neurons in rat brains. FMRP co-fractioned with polysomes in the rough ER in human cell lines and mouse brain. Immunoreactivity of FMRP was high in neuronal perikarya, part of rough ER rich in ribosomes near the cisternae. FMRP was immunolabelled in dendritic spines (Feng et al., 1997). These localization patterns contributed to the understanding of the normal function of FMRP, specifically nucleocytoplasmic shuttling and somatodenritic ribosomal association. The presence of localization signals in FMRP suggested that FMRP may shuttle between nucleus and the cytoplasm. The presence of RNA- binding motifs and association with translating ribosomes in an RNA-dependent manner implicated FMRP in protein translation. Absence of a missense mutation, 1304N in FMR1, was detected in fractions containing translating ribosomes, indicating the absence of FMRP-polyribosome association (Feng et al., 1997). We further wanted to know if the observed RNA binding was selective and an intrinsic property of FMRP. FMRP was shown to exhibit selectivity for binding poly(G). Recombinant isoforms lacking the RGG box and with intact KH domains showed weak interaction of FMRP with RNA. When the amount of FMRP was increased, binding with RNA also increased demonstrating the intrinsic property of FMRP to selectively bind RNA (Brown et al., 1998).
Transcriptional silencing of FMRP, because of expansion of a CGG repeat, implicated both DNA methylation and histone deacetylation in the FXS mechanism. We proposed a mechanism whereby methyl cytosine-binding protein MeCP2 recruits histone deacytelases to methylated DNA , resulting in histone deacylation, chromatin condensation and transcriptional silencing. Acetylation of FMR1 was indeed shown to be decreased in FXS pateints. Inhibition of methylation increased the association of acetylated histones with FMR1 in patients. Conversely, increased inhibition of histone deacetylases in FXS patients increased FMR1 DNA association with histones. However it did not result in transcription activation (Coffee, Zhang, Warren, & Reines, 1999).
Model Systems of FXS
Knockout mouse models
We designed a knock out mouse model for FXS by the homologous recombination of target vector pMG5 (5.7 kb mouse genomic FMR1 DNA). We interrupted exon 5 using a neocassette and introduced it to embryonic stem cells. Western blot analysis showed that no FMRP was produced. Testicular weight was higher in the knock out model compared to normal mice (macroorchidism). Exploratory behavior, tested through the two-chambered light-dark transition test by the number of beam crossings, was also high in knockout mice compared to normal littermates. Cognitive deficit was also seen in knockout mice compared to normal littermate through the Morris water maze test. All these physical and behavior tests were of deficits exhibited by FXS patients. Therefore we successfully created the first knockout mouse model of FMR1 in 1994 (Bakker et al.). Failure in the Morris water maze test, implicated spatial memory as being a specific cognitive damage and therefore we became interested in looking at the hippocampus and long term synaptic plasticity. Other scientists started looking at the effect of FMR1 knockout on dendritic spines in the visual cortex (Comery et al., 1997). Knockout mice showed increased dendritic spines compared to WT (as seen in Figure 1). It also showed increased incidence of long, thin dendritic spines. This long, thin dendritic spine phenotype is that exhibited during early synaptogenesis in the developing brain. Therefore it is implied that knockout have a reduced tendency to mature into adult morphology. Increased spine density implied that knockout mice go through impaired pruning.
We examined the knockout mice using the conditional fear paradigm. The knockout mice displayed significantly less freezing behavior than their WT littermates following both contextual and conditional fear stimuli. These data suggested that amygdala deficit may also be involved in FXS (Paradee et al., 1999).
We also constructed a knockout mouse model for FXR2, one of FMR1 homologs. FXR2P shows high sequence similarity to FMRP and share similar functional domains that are implicated in RNA binding. This knockout mice was constructed by inserting an antisense neocassette in exon 7 to remove exon 7. FXR2 knockoout did not express FXR2P. Several FXS-typical behavior tests were done on this knockout mice and showed deficits successfully. The knockout mice showed aberrant hyperactivity, low motor coordination, low sensorimotor gating, deficient freezing response, decreased sensitivity to heat stimulus and deficient cognitive abilities (Bontekoe et al., 2002).
Drosophila model
We developed a Drosophila FXS model using loss- of-function deletion mutants and overexpression of the FMR1 homolog (dfxr). We characterized dfxr gene and found out that it is cytoplasmic and highly expressed in the nervous system. The expression levels were high in optic lobes and low in muscles and neuromuscular junction (NMJ). Overexpressed WT dfxr showed wing postural defect and droop or held-up wings. Therefore we found out that dfxr expression level is critical for normal neuromuscular functions.
The visual system was also analyzed after the overexpression of dfxr. It showed rough eyes, and abnormal photoreceptor pattern. The null mutant and overexpression both showed less synaptic transmission compared to WT littermates.
Therefore we concluded that both increase and decrease in dfxr impaired synaptic transmission in the visual system. In the NMJ, dfxr null mutant, pronounced synaptic overgrowth, elaboration of synaptic terminals, excessive arboreal branching and increase in the number of synaptic boutons was observed. Conversely, when dfxr was overexpressed, synaptic undergrowth was observed and enlargement of single synaptic boutons was observed (Zhang et al., 2001).
We showed that dfxr is expressed in brain neurons but not in glia. We also found out that both gain and loss in function of dfxr function disabled neurite extension, axon guidance and showed irregular branching. Dfxr mutants also displayed strong eclosion failure and circadian rhythm defects (Morales et al., 2002). This data suggested that different neuronal subtypes are affected differentially by the loss of dfxr.
Mechanisms of FXS
Disabled translational regulation
As discussed earlier, FMRP is a RNA-binding protein and FMRP-mRNA complex are associated with translating polyribosomes. Missense mutation, I304N, has shown evidence of prevention of polyribosome association. Therefore the association between FMRP and polyribosomes is functionally important. So we hypothesized that if FMRP levels decrease, mRNAs that associate with FMRP-mRNP complex are translationally misregulated, which might lead to cognitive deficits. We used microarrays to identify mRNAs that are selectively associated with FMRP-mRNP complexes in mouse and identify mRNAs that exhibit abnormal polyribosome profiles in FXS patients. By comparing these two sets of mRNAs, we identified a subset of mRNAs that associate with FMRP in vivo and display abnormal polyribosome profiles in the absence of FMRP. Nearly 70% of those transcripts contained a G quartet structure, demonstrated as an in vitro FMRP target. Therefore we concluded that translational dysregulation of mRNAs normally associated with FMRP might be the cause of FXS (Brown et al., 2001).
Since the G quartet structure was demonstrated as an in vitro target, we hypothesized that FMRP RGG box binds to intramolecular G-quartets. Therefore we identified mRNAs encoding proteins involved in synaptic development that harbor FMRP binding elements. Majority of these showed altered polysome association in FXS patients. G-quartets were concluded to serve as physiologically relevant targets for FMRP. Many of the mRNA targets identified were involved in either synaptic function of dendritic growth was consistent with previous findings (Darnell et al., 2001).
Disabled synaptic plasticity
As shown in models of FXS, altered synaptic plasticity is a typical phenotype observed in FXS. However we specifically asked which component of synaptic plasticity was altered in FXS. Our study showed that a form of metabotropic Glutamate receptor (mGluR)-dependent synaptic plasticity, long term depression (LTD) is enhanced in the hippocampus of mutant mice lacking FMRP (as seen in Figure 1). Therefore we speculated that FMRP regulated LTD downstream of the mGluRs, likely at the level of mRNA translation (Huber et al., 2002).
Finding the mechanism by which the loss of FMRP altered synaptic function and eventual mental retardation was our next goal. For that purpose, we used small interfering RNAs against FMR1 to show that a decrease of FMRP in dendrites results in an increase in internalization of AMPA receptor subunit, GluR, in dendrites (as seen in Figure 1). This increase in internalization of AMPAR is caused by excess GluR signaling. We found out that when FMRP levels decreased, the amount of internalized AMPAR increased and the amount of surface AMPAR decreased. This provided the first link between altered AMPAR trafficking and FXS. When the FMR1 was low, translational inhibition through anisomycin decreased the ratio of internalized GluR1 to total GluR1. Therefore translational inhibition blocks GluR1 internalization. Conversely, we showed that the ratio of internalized GluR1 to total GluR1 increases on induction of translation through DHPG when FMR1 is low. In fact, when action potential was blocked using TTX, when FMR1 was low, detected complete rescue in the FMRP-deficient dendrites and the ratio of internalized to total GluR1 decreased significantly (Nakamoto et al., 2007).
Microtubule stability
We were still interested in gaining insight into the question of how does FMRP govern normal synapse development in the brain. As described earlier, mRNA targets were identified that were involved in synaptic function of dendritic growth and one of those were microtubule associated protein 1B (MAP1B) (Darnell et al., 2001). We hypothesized that FMRP expression represses the translation of MAP1B and that FMRP was required for the decline of MAP1B during synaptogenesis in neonatal brain development. We found out that in knockout mice, when FMRP levels are low, MAP1B translation is misregulated, indicated by increased and more active polyribosome association. Nocodazole treatment decreases microtubule polymerization and increases microtubule depolarization and consequently increased acetylated alpha-tubulin. Low levels of FMRP also lead to delayed MAP1B decline and elevated MAP1B levels. This is caused by abnormally rigid and stable microtubule, indicated by the increased resistance of microtubules to nocodazole treatment in knockout mice. This increased resistance to microtubule depolarization showed that microtubule stability is abnormally high in FXS (Lu et al., 2004). Increase in MAP1B is caused by FMRP-dependent loss of translation suppression and therefore aberrant microtubule dynamics. And so FMRP can be implicated in cytoskeleton organization during development and abnormal microtubule stability can be attributed to FXS pathology (as seen in Figure 1).
RNA-mediated neurodegeneration
We just discovered a novel neurodegenerative disease insome permutation carriers of FMR1 alleles. These premuatation carriers have an intermediate number of CGG repeats. Interestingly, no neurodegeneration has been observed in FXS patients, who do not express FMR1, and therefore we hypothesized that expanded rCGG repeats of the permutation transcript may lead to neurodegeneration. The model system we used was fruit flies, where we showed 90 rCGG repeats alone were sufficient to cause neurodegeneration. This neurodegeneration exhibited neuronal inclusion bodies rich in heat shock protein 70 (Hsp70). We therefore provided the first in vivo experimental demonstration of RNA-mediated neurodegeneration (Jin et al., 2003).
Therapeutic Insights
The discovery of molecular targets of FXS gave us insight to possible therapy options that could alleviate certain aspects of FXS pathology. These include inhibition of actin polymerization, GABA agonists, mGluR antagonists, CGG repeat- binding proteins and overexpression of Hsp70.
Inhibition of actin polymerization
PAK is a serine-threonine kinase that plays a role in actin polymerization and dendritic morphogenesis. When PAK was inhibited through a (dn)PAK transgene in the forebrain of mice, dendritic spine density decreased, long and thin spines’ density decreased, and long-term potentiation was enhanced (as seen in figure 2). All these phenotypes show a rescue of FXS pathology (Hayashi et al., 2007).
We then tried to look at the pathway of therapy by asking if PAK and FMR1 antagonize each other to regulate spine morphology and synaptic function. For this purpose, we genetically inhibited PAK and crossed dnPAK and FMR1 knockout mice. From the offspring generation, we analyzed WT, FMR1 knockout, dnPAK and double mutant. As expected, we found out that spine density and length decreased in double mutant compared to FMR1 knockout. LTP, determined by the frequency of excitatory post-synaptic potential, of double mutant mice was similar to WT. Rescue of behavioral abnormalities was also observed as double mutants showed similar behavior patterns as WT compared to dnPAK and FMR1 knockout. These behavioral rescue patterns include rescued hyperactivity, stereotypy and hypoanxiety (Hayashi et al., 2007).
GABA agonists
One of the lethal phenotypes of FXS (as seen in Figure 1) as described earlier is excess glutamate signaling. We screened a chemical library of 2,000 compounds and identified nine molecules that rescued this pathology. Three of those nine were implicated in the GABAergic inhibitory pathway. Therefore we wanted to see the effect of GABA treatment as a potential therapy. We indeed found out that GABA treatment rescued the mushroom bodies defects, excess translation and abnormal courtship behavior. GABA treatment led to the inhibition of the enhanced excitatory pathway in FXS (Chang et al., 2008) (as seen in Figure 2).
mGluR antagonists
The correction of FXS phenotypes by genetic and pharmacological reduction of mGluR signaling is developing as the most promising therapy for FXS. We addressed the targeting of intracellular signaling pathway. PI3K is a key signaling molecule downstream of mGluRs. Our study has shown than PI3K activity is significantly increased in FMR1 knockout. We hypothesized that regulating PI3K activity and signaling can regulate FMRP levels. We therefore substantiated that PI3K antagonist treatment could rescue the phenotype of FMR1 knockout in hippocampal neurons. Our hypothesis was supported and we found out that indeed the number of internalized AMPAR and dendritic spine density decreased (Gross et al., 2010) (as seen in Figure 2). Therefore inhibition of PI3K is promising therapy for FXS.
CGG repeat-binding proteins
rCGG repeat-binding proteins (RBP) have been shown to be sequestered from their normal function by rCGG binding in FXTAS neurodegeneration. We identified two known CGG repeat-binding proteins (RBP), Pur a and hnRNP A2/B1 as they were previously shown to associate with permutation-length rCGG repeats. We showed the interaction of Pur a and rCGG repeats and that it is conserved and sequence-specific. We found out that overexpression of Pur a in flies suppressed rCGG- mediated neurodegeneration in a dose-dependent manner (Jin et al., 2007).
Hsp70 overexpression
Hsp70 is a molecular chaperone found in the inclusions in the RNA-mediated neurodegeneration. Studies in both mouse and flies have shown that overexpression of Hsp70 in- crease resistance to polyglutamine-induced toxicity (Jin et al., 2003).
Conclusion
Throughout the twenty-year of research in my lab and in others worldwide working on FXS, we have answered cru- cial questions regarding the pathology, etiology, mechanism and therapy of FXS. However we are still looking for more mechanis- tic insight into FXS pathology and therapeutic understanding of FXS. Eventually we will have to find a cure.
Finding the molecular basis of FXS through studying its underlying mechanism can not only help FXS patients world- wide, it can also give us insight into other broader diseases like autism. Therefore studying the pathophysiology of FXS is im- portant and we are still struggling to get the final answers.
Biological Chemistry, 273(25), 15521-15527.
Brown, V., Darnell, J. C., O’Donnell, W. T., Tenenbaum, S. A., Jin, X., Feng, Y., … & Darnell, R. B. (2001). Microarray identification of FMRP-associated brain mRNAs and al- tered mRNA translational profiles in fragile X syndrome. Cell, 107, 477-487.
Chang, S., Bray, S. M., Li, Z., Zarnescu, D. C., He, C., Jin, P., & Warren, S. T. (2008). Identification of small molecules rescuing fragile X syndrome phenotypes in Drosophila. Nature Chemical Biology, 4(4), 256-263.
Coffee, B., Zhang, F., Warren, S. T., & Reines, D. (1999). Acetylated histones are associated with FMR1 in normal but not fragile X-syndrome cells. Nature Genetics, 22(1), 98-101.
Comery, T. A., Harris, J. B., Willems, P. J., Oostra, B. A., Irwin, S. A., Weiler, I. J., & Greenough, W. T. (1997). Abnormal dendritic spines in fragile X knockout mice: maturation and pruning deficits. Proceedings of the National Acade- my of Sciences, 94(10), 5401-5404.
Darnell, J. C., Jensen, K. B., Jin, P., Brown, V., Warren, S. T., & Darnell, R. B. (2001). Fragile X mental retardation protein targets G quartet mRNAs important for neuronal function. Cell, 107(4), 489-499.
Feng, Y., Gutekunst, C. A., Eberhart, D. E., Yi, H., Warren, S. T., & Hersch, S. M. (1997). Fragile X mental retardation protein: nucleocytoplasmic shuttling and association with somatodendritic ribosomes. The Journal of Neurosci- ence, 17(5), 1539-1547.
Fu, Y. H., Kuhl, D., Pizzuti, A., Pieretti, M., Sutcliffe, J. S., Rich- ards, S., … & Caskey, C. T. (1991). Variation of the CGG repeat at the fragile X site results in genetic instability: resolution of the Sherman paradox. Cell, 67(6), 1047- 1058.
Garber, K. B., Visootsak, J., & Warren, S. T. (2008). Fragile X syndrome. European Journal of Human Genetics, 16(6), 666-672.