Ant Diversity in the Urban Mosaic
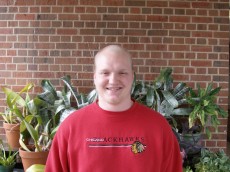
Jeremy Boeing
Department of Biology
Lake Forest College
Lake Forest, IL 60045
Abstract
Cities are the fastest growing environment in the world today. As cities increase in size, they disturb and destroy the sur- rounding natural environment. Traditionally, urban centers have been thought of as a homogenized environment supporting a few wide- spread species. However, I hypothesize that variation in city environ- ments plays a major role in biodiversity and species assemblages. I use ants as a representative species to address microenvironments in the city. I discovered that microenvironments in the urban mosaic have unique communities and that both microenvironment area and isolation play a role in determining species assemblages. This study exhibits the importance in understanding complexity in urban envi- ronments and may help us limit negative effects of human expansion on other organisms.
Chapter 1 – Understanding Urbanization
An Introduction to Urban Ecology
Urban Ecology has come to the forefront of ecological studies in an attempt to further understand what patterns of biodiversity are present within urban environments. Urban Ecology looks to use the many princi- ples of ecology and apply them to the ever expanding cityscape to un- derstand the roles the physical environment in urban landscapes play on species assemblages (Pickett et al. 2008).
Cities are the fastest growing environment of the world today (McIntyre 2000, Pickett et al. 2008). The Global Rural-Urban Mapping Proj- ect has shown that urban environments make up more than 3 % of the world’s land surface (Ciesin 2011). In addition to the large spatial extent of urban areas, human populations are clustered in urban environments. According to the 2010 U.S. census, over 71% of the American population may be found within heavily urbanized territories. Despite this knowledge, very little is known about how non-human species interact with urban en- vironments (McIntyre 2000, McKinney 2002, McDonnell and Hahs 2008, Pickett et al. 2008).
Traditional ecological thought has treated cities as homogenous species poor environments that destroyed natural areas as they expanded (McIntyre 2000). With further investigation into these “concrete jungles” we may find that instead of a single species poor environment, that there is a mosaic of microenvironments with diverse species assemblages. Ur- ban environments often have a few pockets of untouched natural environ- ment. Additionally, many cities incorporate a large degree of “green space” through the use of gardens and parks. To fully address large cities ecologi- cally, we need to address both city environments as well as their effects on the natural environment they have altered (Pickett et al. 2008).
The study of organisms and how they interact with their environ- ment has always been a core component of ecology. The study of ecolo- gy has been subdivided into several different fields, including ecosystem ecology, community ecology, population ecology, and organismal ecology, each addressing a unique environmental scale. The largest of these scales addresses the world. The planet is classified into large units called biomes. Each biome helps categorize the various regions of the Earth’s ecosys- tems based upon the climate and vegetation of the area. Within these bi- omes, different communities survive and interact with one another (Morin 2009). These various communities are addressed in community ecology which focuses on how different populations interact with one another (Mo- rin 2009). Population ecology focuses on the many individuals within these population and how they interact with other individuals in that same popu- lation as well as the environment (Hawley 1950). Each level of ecology al- lows new insights into organisms and their interactions in the natural world. However, ecological studies have traditionally been restricted to natural environments (McIntyre 2000, Pickett et al. 2008).
Despite the many ecological principles we have defined in natural sys- tems, little has been done to understand what principles act on species in urban environments (Blair 1999, McIntyre 2000). Urban environments,
for instance, are the only environment that occurs in every biome on the planet. In addition, it is the fastest growing environment worldwide (Svire- jeva-Hopkins and Schellnhuber 2006, Martine and Marshall 2007). In light of the expansion of urban environments and the increased presence of non-human species in cities, ecologists have begun to explore the ecolog- ical pressures of cities in the relatively new field of urban ecology (Pickett et al. 2008).
To understand the ecological patterns and pressures within an environment, cities notwithstanding, organisms are classified into a Lin- naean hierarchy. The smallest units used in ecology are species. Organ- isms may be classified into species using various concepts. The most com- monly used species concept in ecology is based on reproductive isolation (Agapow et al. 2004). If two organisms cannot produce viable offspring, then they are considered two separate species (Palumbi 1994, Schluter 2009). Using this species concept, ecologists can examine how ecological pressures affect the diversity in both natural and urban environments.
In order to address the diversity of environments, the term biodiversity was coined by W.G. Rosen in 1985. Biodiversity is used to describe the degree of variation of life on the planet. Species distributions and biodiversity are intertwined. Many of the natural environments that have been observed and defined are now broken up by large urban environments that have sprung up over time (Gibb and Hochuli 2002, Coy 2006). In light of shrink- ing natural environments and the increase of urbanized environments, it has become increasing important to understand what role urban environ- ments play on biodiversity. Cities provide different environmental condi- tions than similar rural environments (White and McDonnell 1988, Hogs- den and Hutchinson 2004). The addition of traffic, pavement, and human interactions may affect how species interact with one another and in turn alter the biodiversity of urban environments (Connell 1961, Hersteinsson and Macdonald 1992).
Species Interactions
Species interactions help to determine both species distributions and community structures (Tylianakis et al. 2008). A mutualism occurs when two species positively influence one another. Nitrogen fixing bacteria found in the roots of many plants provide one key example. The bacteria are protected from the environment by using the plants roots as housing, and in return provide the host plant with nitrogen the plant cannot acquire on its own (Simms and Taylor 2002, Pinto-Tomás et al. 2009). In urban systems, nitrogen levels are reduced (White and McDonnell 1988). There- fore, plants with the nitrogen fixing mutualism are more likely to succeed in urban environments (White and McDonnell 1988).
Commensalism positively influences one species while the oth- er goes unaffected. Spiders spin their webs across two different plants. Though the web will allow the spider to acquire food, the plant receives no benefit from acting as a support. Urban environments add a new level of human commensalism. For instance, house sparrows are common in man-made habitats as they use man-made structures as shelter from other environmental conditions (Saetre et al. 2012).
Additionally, and most commonly in the world, competition oc- curs when two species are negatively affected by the interaction. Several types of competition may occur but usually involve two species fighting for control of a resource (Tilman 1977, Goldberg 1990). Competition, though quite common in natural environments, may be enhanced in urban envi- ronments due to lower resource availability for a number of different or- ganisms (Rebele 1994, Pickett et al. 2008). Using species interactions, we may expand our understanding of the urban environment.
Equilibrium Theory and Habitat Fragmentation
Resource availability may enhance the competitive interactions between species. One of the many resources organisms require is area. Area theory claims that the larger the land mass, the more species that land mass may support (MacArthur and Wilson 1963). Area is one of the many abiotic factors that may help explain the biodiversity found on a land mass and has been shown to effect species assemblages across a va- riety of different environment types (MacArthur and Wilson 1963, Bolger et al. 2000, Gibb and Hochuli 2002, Carpintero and Reyes‐López 2014). Shortly after the theory was proposed, it was applied to continents to help explain the number of species seen over long time spans (Marshall et al. 1982).
In addition to area, isolation has been shown to influence the diversity of islands and other habitat systems (Rice 1987, Palumbi 1994, Carpintero and Reyes‐López 2014). As the isolation of a land mass in
creases, the number of species on that land mass is predicted to decrease (MacArthur and Wilson 1963). The ability of organisms to reach these land masses depends are their ability to disperse.
Species that show a high dispersal ability, are expected to be found on islands that may be considered isolated for many other species (Higgins and Richardson 1999, Rehage and Sih 2004). For example, birds tend to travel great distances thanks to flight. Thus, if a species of bird is found on one island, we would expect it to be found in other islands of the same type even if those islands are relatively far away. On the flip side, a small beetle cannot travel the same great distances that birds may. Even if an island of similar type occurs nearby, the beetle may have no way of traveling between fragments. This simple juxtaposition shows how the as- semblages of the two islands could be affected.
To address area and isolation together, Wilson and MacArthur (1963) proposed the equilibrium theory of island biogeography as a way to predict diversity. This theory was initially created to address diversity observed on island chains. The theory claims that islands, which are sepa- rated by large quantities of water that terrestrial organisms cannot inhabit, have different areas and thus support different numbers of species (MacAr- thur and Wilson 1963). As the area of the island increases, the diversity on that land mass should also increase. In addition, the distance from one island to its nearest neighbor helps ecologists understand how isolation affects species assemblages (Higgins and Richardson 1999, Soons and Bullock 2008). As previously mentioned, organisms vary in their ability to disperse, thus the separation of islands affect organisms differently (Re- hage and Sih 2004). These principles of equilibrium and area theory have since been applied to habitat fragmentation in the field of conservation (Simberloff and Abele 1982, Saunders et al. 1991).
Habitat fragmentation results when the natural environment is broken into smaller pieces that are separated from one another. The re- maining pieces of natural environment are thus referred to as habitat frag- ments. Area theory has been applied to natural environments that have been fragmented by a variety of factors. It has been shown that, similar to islands, the larger the habitat fragment, the greater the number of species observed within that fragment (Herkert 1994, Nufio et al. 2009). This idea may also apply to environment types that exist within urban systems. Green spaces like parks, green rooftops, and even backyard gardens provide dif- ferent green “islands” within the cityscape that organisms may use. Instead of being separated by water, the green spaces within the city are separated by matrix habitat comprised of impervious concrete on which organisms cannot colonize or survive. This results in isolated differently sized green spaces. Similarly, green spaces and habitat fragments maintain their own degree of isolation (Bolger et al. 2000). Some natural fragments exist close together and may allow organisms to easily move between them, while others are not close together and thus difficult for organisms to disperse to (Bunn et al. 2000). However, this type of fragmentation also introduces a new level of complexity not seen before with island systems.
In habitat fragments, the assemblages found at the edge of hab- itat fragments do not mirror the assemblages found in the inner area of the fragment (Murcia 1995, Woodroffe and Ginsberg 1998). This phenomenon is due to edge effects. It is hypothesized that the inner area of fragments provides a different physical and biotic environment than the outer edges of fragmented habitats due to the mixing of two different environments lead- ing to the differences observed between the edge and inner assemblages (Murcia 1995). Smaller habitat fragments may be affected by edge effects much more than larger natural habitats (Bolger et al. 2000). Within cit- ies, the many small man made green spaces may be more prone to edge effects than larger fragments of natural habitat (Bolger et al. 2000). The edge of habitat fragments may act as a gradient between environments as the external environment slowly blends into the fragmented environment (Gehlhausen et al. 2000). This would allow species from the external envi- ronment to penetrate some degree into fragmented environments resulting in a new edge assemblage. For urban systems, instead of seeing different assemblages near the edge of an environment, species diversity may de- crease with increasing proximity to the edge of the fragment.
The fragmentation model becomes more complex when the idea of population size is added to the mix. For populations to survive, some degree of genetic variation must exist in a population (Young et al. 1996). If not, population bottlenecking may occur and result in serious genetic is- sues within a population. Mutation provides some genetic variation but may not be enough on its own to ensure population stability over long periods of time in small populations (Lenormand 2002). In particular, urban environ- ments have proved detrimental to the genetic variation of species (Young
et al. 1996, Hitchings and Beebee 1997). To reduce bottlenecking effects, some amount of gene exchange with other populations is necessary to ensure the continued success of an established population (Lenormand 2002). This genetic exchange is often a result of immigration and emigra- tion from a habitat to a new location (Lenormand 2002).
When applied to the fragmentation concept, the source-sink model of fragmentation arises (Amarasekare and Nisbet 2001). This model argues that there are some species rich fragments and other species poor fragments. The species rich fragments allow increased genetic variation and sustainable populations, while sink fragments show increased extinc- tion rates and fluctuating populations (Amarasekare and Nisbet 2001). The source fragments provide the sink fragment with an influx of genetic diversity as individuals migrate from source populations to sink popula- tions. This allows both populations to continue uninhibited by genetic is- sues while maintaining current species assemblages (Amarasekare and Nisbet 2001). Migration rates will vary based upon the distance from a sink to the nearest source fragment (Higgins and Richardson 1999, Soons and Bullock 2008). Research into urban habitats has shown that sinks help stabilize metapopulations (Foppen et al. 2000). It has also shown that when habitat fragments are isolated, as is often the case when urban en- vironments expand into previously undisturbed areas, the genetic diversity of species tend to decrease with the age of the fragment (Hitchings and Beebee 1997). It is hypothesized that the outlets provided by sinks helps to stabilize source populations and allows species to persist for a longer period of time (Foppen et al. 2000). Many genetic problems that occur in cities are thought to be due to isolation and lack of dispersal.
Isolation and Connectivity
Conservation efforts have focused on solving the isolation co- nundrum by investigating a variety of ways to connect fragments (Haig et al. 1998, Hodgson et al. 2009). Connectivity between natural environments changes over time. Biogeographically, land bridges allowed several differ- ent species to cross into previously isolated continents (Simpson 1940). Land bridges are categorized into two different groups depending on their degree of selectivity (Simpson 1940). The first group is called corridors, a connection between two isolated fragments or land masses that allow all species across (Simpson 1940). Additionally, corridors maintain the same habitat type as the fragments. For example, two separate fragments that are heavily forested are connected by a thin strip of forest habitat. This habitat should allow all the species in each fragment to freely move across to the new area. If the bridge connecting different environments is truly a corridor, we expect to see similar species assemblages on both sides. Within urban environments, many areas such as rivers and railways con- nect several different types of green space. These different land types act as urban corridors connecting previously isolated areas in the city.
In other instances, the connection may act as a filter bridge. Filter bridges allow species to cross between fragments but select for particular traits (Simpson 1940). An example of a filter bridge is provided by the migration of cichlids from South America up into Central America. Cichlid species that could survive increased salinity were able to use the connection while those with normal tolerances could not (Říčan et al. 2013). The signifi- cance filter bridge connections within urban environments may significantly reduce the consequences of isolation on population genetics in urban sys- tems (Foppen et al. 2000).
Both dispersal ability and connectivity play significant roles in determining the species distributions in cities. Cities provide new disper- sal vectors that are now being used by some organisms (Von der Lippe and Kowarik 2008). Plants and small species may hitch a ride on vehicles passing through the cityscape allowing them to expand their range from city centers out into more rural environments (Von der Lippe and Kowarik 2008). However, this pattern seems to be limited to smaller species (Von der Lippe and Kowarik 2008). For larger species like mammals, dispersal between green spaces within urbanized areas does not happen as often as in natural habitats (Harris and Trewhella 1988). This lack of dispersal may prove detrimental to small populations in urban systems which rely on the movement of organisms for continued survival. In general, low frequencies of dispersal tend to indicate that the environment maintains little connectiv- ity (Bunn et al. 2000). Researchers have begun to focus on environments like river ways that allow species to disperse to other green spaces within urban zones (Säumel and Kowarik 2010).
To further improve the connectivity of urban systems, urban gre- enways (artificially constructed strips of greenery and forest) have been built in many cities to connect both cities to the surrounding rural areas as
well as isolated urban green spaces (Schiller and Horn 1997). The ecolog- ical impact of greenway use has been shown to improve species mobility within cities and may therefore improve population interactions and reduce detrimental genetic effects (Schiller and Horn 1997). However, many ex- ternal factors may affect the impact greenways have on species (Schiller and Horn 1997). It is also worth mentioning that many of the species that are dispersed across these connective urban environments are invasive species (Säumel and Kowarik 2010).
Invasive Species
Invasive species, which are thought to be more common in ur- ban systems, are non-native species that are introduced through some hu- man mediated dispersal vector (Rejmánek and Richardson 1996, Phillips and Shine 2006). Once present, they are considered a pest to humans and usually negatively affect the native fauna (Tsutsui et al. 2000, Pimentel et al. 2005). Species with the ability to establish in one introduction, gener- alize on many resources, and reproduce frequently and in great numbers are at a higher risk to become invasive in a new environment (Rejmánek and Richardson 1996). Once present, invasive species can cause seri- ous changes to the species already present in an environment (Tsutsui et al. 2000, Phillips and Shine 2006). Cane toads, which were introduced to Australia, have caused serious changes in predator activity and much of their success may be attributed to urban environments and the roads that connect them (Brown et al. 2006, Phillips and Shine 2006, Urban et al. 2007). In many cases, invasives arise where they can outcompete the na- tive fauna (Rejmánek and Richardson 1996). Environments may be more susceptible to invasive species based upon their diversity and disturbance (Rejmánek and Richardson 1996, Tsutsui et al. 2000, Urban et al. 2007). The idea of biotic resistance applies to environments with high species diversity that do not give invasives enough resources or niche space to become established (Rejmánek and Richardson 1996, Levine et al. 2004). Within urban environments the species diversity of many native organisms is lower than in surrounding natural areas (Angold et al. 2006). With the lower species diversity in urban environments they are likely to have lower biotic resistance to invasion than surrounding natural environments. When the low biotic resistance is paired with the high degree of disturbance found within cityscapes, urbanized areas become prime locations for invasive species persistence (Urban et al. 2007).
Urban environments are at further risk of invasives due to hu- man mediated dispersal (Wichmann et al. 2009). Though human mediated dispersal may allow organisms within urban environments to expand out to rural areas, it also allows the introduction of invasive species to urban- ized zones (Ward et al. 2005). One excellent example of urban invasive ecology is the spread of the Emerald Ash Borer in North America (Poland and McCullough 2006). This introduced species has quickly decimated ash tree populations across urban forests in the United States (Poland and McCullough 2006). It has been continuously spread by human beings transporting firewood and wooden pallets (Poland and McCullough 2006). Many other invasive species may also be unintentionally spread to cities when trees are placed in new parks or gardens (Pimentel et al. 2005). The presence of invasive species within urban zones not only comes at a high ecological cost, but also a high economic cost (Pimentel et al. 2005). In the United States alone, over 50,000 invasive species are present costing the United States approximately $120 billion a year (Pimentel et al. 2005). This money is spent both to reduce the ecological impact that invasive species may have as well as attempting to prevent their further spread (Pimentel et al. 2005).
Urban History
City design has adapted overtime and modern cities look en- tirely different than the urban areas of the past. Cities have traditionally been planned around the needs of working people (Cervero and Kockel- man 1997, Montgomery 1998). As such they were designed in a way that maximized travel so that individuals could easily traverse the landscape (Cervero and Kockelman 1997). The demand to easily travel and reach destinations as quickly as possible lead to the development of elevation leveling and paving of streets across the cityscape (Cervero and Kockel- man 1997). In addition, compaction came to the forefront of city design. This means that early cities tried to eliminate unnecessary space and put shops and buildings as close together at possible (Cervero and Kockelman 1997, Montgomery 1998). This eliminated green spaces within cities as they took up space that could easily be filled by another building or shop. The lack of green space within urban environments caused temperatures
to rapidly rise in cities (Oke 1973). Without green space, incident sunlight that hit cities was absorbed into tar or reflected by the concrete of buildings. The dispersion of this sunlight greatly increases the heat in urban systems resulting in what scientists call the urban heat island effect (Oke 1973). Additionally, increased mental illness in cities has been linked to the lack of green space, and the addition of green space is believed to help minimize depression in city residents (Montgomery 1998, Jackson 2003, Peacock et al. 2007). Not to mention that limited green space is believed to drastically reduce the wildlife present in urbanized areas (Bolger et al. 2000, Peacock et al. 2007). These factors influenced the incorporation of green space into the urban environment (Cranz 1982, Jackson 2003). Shortly after heavy urbanization, the pattern of including green space within cities was fully incorporated into urban design (Cranz 1982, Montgomery 1998).
Green space was incorporated into urban areas as either pre- serving the fragments of natural habitat that remained, or creating urban parks, adding trees to street medians, and most recently green rooftops. The benefits of green space within cities for both humans and other or- ganism are undeniable (Oke 1973, Montgomery 1998, Kark et al. 2007). Initially, these green spaces were intended to increase the aesthetic appeal of cities to the general population (Cranz 1982). Parks, for instance, al- lowed for the increase in recreational activities and leisure for city residents (Cranz 1982). However, they also reduce the magnitude of the heat island effect in heavily urbanized zones by absorbing and converting sunlight in- stead of reflecting it back into the urban environment (Oke 1973). Further reducing the urban heat island effect became a priority as trees and shrubs lined street medians, and parks included urban gardens to limit impervious surface lowering urban temperatures (Cranz 1982). It was shortly after the incorporation of these green spaces that the field of urban ecology began to investigate the effects urbanization had on species assemblages.
With a new understanding of the role urbanization plays in ecol- ogy, another alteration of cities has taken place. Parks, gardens, and even street medians provide environments that many small organisms could in- habit. As mentioned earlier some cities have begun incorporating urban greenways into their design (Schiller and Horn 1997). These greenways provide additional green space connectivity instead of just relying on rail- ways, street medians, and other unintentional connective environments. Though a new and ecologically beneficial addition to cities, greenways have not been the most popular addition to the cityscape due to its use of space (Madre et al. 2013). In addition to producing these greenways, cit- ies have turned to green rooftops because of their economic benefits and minimal use of space (Brenneisen 2006). These rooftops have provided both economic advantages to urban systems and novel habitat for urban species (Bass et al. 2003, Brenneisen 2006, Oberndorfer et al. 2007).
We are only just now beginning to understand the complexities that green spaces in cities have on urban species. By viewing the green spaces in cities through an ecological lens, several well-defined ecological principles may be applied to predict and explain which species will succeed in these new environments. Understanding how to maximize the benefits of cities for humans while limiting the impact on wildlife has become a goal of ecologists and urban planners alike. There is no doubt that cities will con- tinue to expand in the future (Ciesin 2011). It thus becomes clear that cities will remain important and need to be understood through urban ecology.
Chapter 2 – Ant Diversity in the Urban Mosaic
Introduction
The United States urban population has risen by over 12% in the last decade (U.S. Census 2010). As such, urban environments are one of the fastest growing habitats in the world (Ellis and Ramankutty 2008). De- spite the rapid growth of urban environments, they remain one of the least studied ecosystems in the scientific literature (McIntyre 2000, McDonnell and Hahs 2008, Schluter 2009). Typically, papers address urban centers as a homogenous environment that contains poor overall species diversi- ty, comprised mainly of human commensals (Blair 1999, McKinney 2002). However, by classifying cities as a single homogenous environment, the full variation of the urban ecosystem is not adequately addressed. As the size of an organism varies, so too must the spatial scale of a study (Wiens 1989). This means that categorizing urban environments as one unit may sufficiently predict patterns for larger organisms, but does not account for small species that colonize and survive in urban environments (McIntyre 2000, Matteson et al. 2008). For these small organisms, cities may instead be addressed as urban mosaics comprised of several microenvironments (Matteson et al. 2008, Menke et al. 2011, Savage et al. 2014).
On a macro scale, habitats in urban centers are traditionally di
vided into two basic groups, fragments of natural areas such as parks and impervious concrete surfaces. However, within the urban center smaller pockets of natural environments survive and relatively little research has been done to understand animal communities in these areas (Rebele 1994, McIntyre 2000). For example, corridors along rivers and transit rail lines are thin strips of remnant habitat or restored landscapes that attempt to mimic surrounding natural environments (Oberndorfer et al. 2007). Back- yards, gardens, green rooftops, and even small green strips running along street medians all bear some similarity to natural environments and provide green space for wildlife (Bolger et al. 2001, Brenneisen 2006, Smith et al. 2006). These microenvironments go largely unnoticed while conservation efforts focus mainly on large green spaces (Bolger et al. 2000, McIntyre 2000, Carpintero and Reyes‐López 2014). Though these small micro- environments may seem inconsequential, they may be used by different organisms as both a corridor system for traversing urban centers, and for habitat in an otherwise simple environment (Bolger et al. 2001, Matteson et al. 2008, Menke et al. 2011, Savage et al. 2014).
Urban microenvironments provide some degree of green space, but their ability to do so is limited by human disturbance (Savage et al. 2014). Common disturbances that occur in urban microenvironments range from mowing the lawn and tree trimming to simply weeding a garden (Schippers and Joenje 2002, Maalouf et al. 2012). Microenvironments oc- cur in different locales resulting in different degrees of human disturbance (Hogsden and Hutchinson 2004).
As such, microenvironments themselves may be categorized by where they fall in this disturbance gradient (Savage et al. 2014). For instance, many green rooftops are planted specifically to mimic the natu- ral environment that once existed there (Brenneisen 2006, Oberndorfer et al. 2007). These rooftops are planted once, watered and then left largely unattended and experience little to no human disturbance or interference. Green rooftops are usually small (restricted by the size of the building they are placed on) and surrounded by cement roofs and other buildings (Bren- neisen 2006, Oberndorfer et al. 2007).
Moving up the disturbance gradient, parks and backyards supply small organisms with a microenvironment that varies from the more natu- ral green rooftops (Matteson et al. 2008). Rather than complex tall grass prairies, parks and residential yards tend to be more simplistic open envi- ronments similar to open savannas predominately composed of manicured grass with only small scattered pockets of natural vegetation containing shrubs, flower gardens, and sparse trees unable to create an overstory. These parks and yards tend to have a higher frequency of human dis- turbance that prevents them from mimicking the natural grasslands. Also, these environments tend to be much larger than a green rooftop or street median (Cranz 1982). Parks are normally surrounded by small additional green space like grass fields or small gardens adding to the green space available for organisms (Cranz 1982, Carpintero and Reyes‐López 2014). At the highest degree of disturbance are street medians. Though appear- ing vertically complex these microenvironments contained homogenized flora, and are constantly maintained, trimmed, and disturbed. Additionally, street medians are linear and corridor-like in nature; this restricts them to a small area surrounded by cement. Green rooftops and parks have much larger areas and are better addressed as isolated habitat fragments. While using this microenvironment approach may not be ideal for large verte- brate species, it may be highly applicable to understanding urban impact on smaller invertebrate species.
Insects are the most diverse group of organisms on the planet and serve many important ecological functions (McIntyre 2000, Holway et al. 2002, Dunn et al. 2007). Within large urban centers, ants may be used to model species assemblage patterns for insects and other small organisms (Bolger et al. 2000, Andersen et al. 2002, Menke et al. 2011). Ants disperse seeds, protect floral species from herbivores, fertilize and maintain the soil, and they are considered an ecosystem engineer (Holway et al. 2002). Additionally, ants are highly visible in the city and commonly associate with humans and their structures (McIntyre 2000). As such, they have been identified as one taxa that directly responds to human induced ecological change (Andersen 1990, King et al. 1998). Ants also adapt and respond to fine grain heterogeneity caused by disturbance (Savage et al. 2014). The association with humans, high abundances, and ability to be easily studied and transported makes ants a prime organism to address differences between urban microenvironments.
As cities continue to expand it becomes critically important to understand how species may use microenvironments that exist within the urban mosaic. Using ants as a representative group, we address two relat
ed questions in this study. First, we will determine if different microenviron- ments within the Chicago urban mosaic contain unique ant assemblages. Second, we determine how the area of different types of microenviron- ments influences ant assemblages. We compare three different microen- vironment types; green rooftops, urban parks, and street medians, which vary in size and disturbance. We predict a decrease in ant species richness as the similarity to natural surrounding flora decreases. Therefore, we pre- dict green rooftops, will have the most diverse ant communities followed by urban parks and finally urban street medians. We predict that as the size of a microenvironment increases, so too should the species richness in that environment and this pattern should be the same for all three microhabitat types.
Methods
Site Information
Chicago is located on the shores of Lake Michigan and is home to over two and a half million people (U.S. Census 2010). As a large urban center, Chicago is also home to a variety of green spaces, including green rooftops, parks, and street medians. Ant communities were sampled from 65 different sites throughout Chicago; eight green rooftops, 12 city parks, and 45 street medians (Fig. 1).
Sampling
All sites were sampled using pitfall traps composed of 50 mL centrifuge tubes, with a 22mm diameter opening. These traps were filled with 25 mL of propylene glycol and 1 drop of non-scented soap to break the surface tension. Green rooftops were each sampled once in June 2012. Parks and street medians were each sampled three times during the sum- mer of 2011, once in June, July, and August. All three sampling periods were combined for analysis.
For green rooftops, the number of traps varied from 6 to 80 traps per rooftop depending on the size of the rooftop, with traps placed every 5m in a grid. In cases where rooftops had soil depths of less than 12.7cm, pitfall traps were cut to shorter lengths. Parks and street medians were sampled by placing five traps spaced 10m apart in a linear transect. On some street medians, where space was limited, traps were placed every 5m. Traps were buried flush with the ground and remained unopened for one day to minimize the effect of disturbance. Traps were then opened for four days.
Pitfall traps were stored at room temperature during sorting. Ants present in each pitfall trap were sorted out by hand and placed in 95% eth- anol for storage. Each individual was identified to the species level using regional species keys (Coovert 2005, Ellison et al. 2012).
Question 1: Unique Ant Assemblages in Microenvironments
Three different techniques were used to characterize the ant communities in different microenvironments. First, a species rarefaction curve was created for each microenvironment comparing the number of species accumulated by the number of traps placed. For ants, the colo- ny acts as the ecological unit, not the individuals captured. To correct for capturing multiple individuals from the same nest, the proportion of pitfall traps in which each species occurred (incidences) was used to quantify species abundance. Second, estimated species richness in each micro- environment was calculated using the Chao 2 index. Third, ANOVA’s were performed on species that comprised more than one percent of the total number of incidences collected to compare the relative occurrences of spe- cies between microenvironments. Both the rarefaction curve and the Chao
2 analysis, were performed using Estimate S v9.0 (Colwell 2005).
A principle coordinates analysis (PCoA) was used to visualize differences in community structure between microenvironment types. Following this analysis, a permutational multivariate analysis of variance (PERMANOVA) was performed to test for differences in ant species incidences, square root transformed, between microenvironment types. A PERMANOVA tests the responses of multiple species simultaneously to the microenvironment factor. The analysis was based on Bray-Curtis dissimilarity with 99,999 per- mutations for each test and pair-wise comparisons. Both the PCoA and the PERMANOVA were performed using PRIMER v6.
Question 2: The Effect of Area on Microenvironments
The effect of area on species assemblages in microenviron-
ments was evaluated in two different ways. First, we used a linear regres- sion to determine the relationship between the log of site area and species richness. We then used a distance-based redundancy analysis (dbRDA) to
determine the relative importance of the log of the total area of the micro- environment and latitude of the microenvironment in explaining ant com- munity variation across all microenvironment types. Since the majority of sampling occurred along a North South transect, latitudinal differences be- tween microenvironments were used as the isolation metric. A dbRDA tests how much of the collected community structure is explained by one vari- able, then it adds a second variable to determine what additional amount of variation is explained. The dbRDA was performed using PRIMER v6.
Results
Question 1: Unique Ant Assemblages in Microenvironments
We captured a total of 10,511 individuals from 23 species; 2,068 individuals representing 9 species were collected from green rooftops, 2,077 individuals representing 17 species from urban parks, and 6,366 in- dividuals representing 22 species from street medians. Species richness for green rooftops, urban parks, and street medians ranged from 1-7, 4-11, and 1-11 species respectively. Eight species were found in all three mi- croenvironment types (Brachymyrmex depilis, Formica pallidefulva, Hypo- ponera opacior, Lasius neoniger, Nylanderia terricola, Solenopsis molesta, Tapinoma sessile, and Tetramorium caespitum). Of these species, Tetram- orium caespitum was the most common occurring at 63 of the 65 sites sampled. Formica montana was found only in green rooftop microenviron- ments. Five species were found only in street median microenvironments (Camponotus discolor, C. nearcticus, Myrmecina americana, Myrmica sp. AF-eva, and M. punctiventris). Myrmecina americana, F. montana, and Myrmica punctiventris were each found at only one site. Two species were
not native to Illinois, T. caespitum and H. opacior.
Rarefaction curves for the number of incidences displayed dif-
ferences in species accumulation as a function of microenvironment type (Fig. 2). Green rooftops accumulated species slower than urban parks (Fig. 2). Street median microenvironments did not significantly differ from either of the other microenvironments as determined by the overlap of the 95% confidence intervals (Fig. 2). All three microenvironments significantly dif- fered in their Chao 2 estimated species richness (Fig. 3). Green rooftops had the fewest species, urban parks were intermediate, and street medi- ans had the greatest estimated species richness (Fig. 3).
Microenvironments significantly differed in area (ANOVA: F1 = 5.128 P = 0.0087). Green rooftops were the smallest microenvironment and ranged in size from 24 to 1,885 m2 (mean +/- SE = 743.9 +/- 227 m2). Urban parks were the largest microenvironment and ranged from 56,448 to 1,481,852 m2 (mean +/- SE = 1,008,210 +/- 679,707 m2). Street medians were an intermediate size and ranged from 138 to 14,960 m2 (mean +/- SE = 1,927 +/- 505 m2). A semi-logarithmic plot displayed a positive linear re- lationship between species richness and microenvironment area (Fig. 4; y = 0.6288ln(x) – 0.0505, R2 = 0.308, F1 = 29.54, P < 0.000001). In addition, a plot of log species richness and log area showed the z-value for our mi- croenvironments to be 0.132. When microenvironment types were tested individually, there was no significant relationship between area and green rooftops (Fig. 5A) or urban parks (Fig. 5B). Species richness did increase with street median area (Fig. 5C; y = 1.5193ln(x) – 5.2543, R2 = 0.5264, F1 = 47.79, P < 0.00001).
Community composition differed as a function of microenviron- ment type (PERMANOVA: P = 0.0215). A pattern with significant centroid separation emerged when the PCoA was analyzed (Fig. 6). Most of the differences in community structure appear to be on the PCO1 axis which explains 45.13% of the variation while there were little differences between sites on the PCO2 axis (Fig. 6). Green rooftops remained fairly restricted to the left side of PCO1 while both parks and rooftops spread fairly evenly across the same axis (Fig. 6). Pairwise analyses between microenviron- ments reveal that green rooftop communities differ from both urban parks (P = 0.0203) and street median (P = 0.016) communities, while ant com- munities in urban parks and street medians did not differ from one another (P = 0.2533).
An analysis of the rank order change between sites from cal- culated similarities in the PERMANOVA revealed that variability between sites within a microenvironment was greatest for green rooftops (39.2% similarity) while urban park sites and street median sites had less variability in community structure (49.8% and 51.3% similarity respectively, Table 1). In addition to low within site similarity, green rooftops also exhibited the lowest between site similarity with urban parks and street medians (Table 1). The SIMPER revealed that the differences between park and street median microenvironment types and green rooftops is largely due to re- duced abundances of all species on green rooftops relative to urban parks
and street medians, especially for Tetramorium caespitum, Lasius neoni- ger, and Solenopsis molesta (Table 2). Though most species decreased in abundance, both Tapinoma sessile and Crematogaster cerasi were higher in abundance on green rooftops compared to the other microenvi- ronments (Table 2). Tapinoma sessile displayed the most robust change and explained around 11% of the dissimilarity between green rooftops and urban parks and street medians (Table 2). Urban parks differed from green rooftops and street medians largely due to the occurrence of Solenopsis molesta within parks (75% of sites) compared to green rooftops (25% of sites), and street medians (47% of sites).
A graph of the relative occurrence of the most common species (comprising at least 1% of the total occurrences) reveals differences in occurrence between microhabitats for five species, Tetramorium caespi- tum, Lasius neoniger, Solenopsis molesta, Lasius flavus, and Tapinoma sessile (Fig. 7). Tetramorium caespitum occurred significantly more often in street median traps than park traps (Fig. 7). Tapinoma sessile, occurred significantly more often at green rooftops and parks and was rarely found in street medians (Fig. 7). Solenopsis molesta occurred significantly more often in urban parks than either of the other microenvironment types (Fig. 7). Both Lasius neoniger and L. flavus rarely or never occurred on green rooftops; as such, they occurred significantly more often in the other two microenvironment types (Fig. 7).
The Distance-based Redundancy Analysis (dbRDA) revealed that area of a site is a significant predictor of community structure (P = 0.001, r2 = 0.08). Adding latitude of a site significantly improved the model and cumulatively explained 12% of the community variation (P = 0.0204, r2 = 0.12).
Discussion
We addressed two questions about how ant communities re- spond to the urban mosaic; first, we determined that different microenvi- ronments contain unique communities, and second, we demonstrated that the area of a microenvironment predicts species assemblages in urban en- vironments. The species accumulation and the estimated species richness of the microenvironments showed increased species richness in parks and street medians compared to green rooftops (Figs. 2 & 3). This could be in part due to the increased species richness associated with the larger areas of these sites (Fig. 4). Ant communities on green rooftops are distinct from urban parks and street medians (Fig. 5). Area also helps to explain the differences in community structure observed between microenvironment types. After accounting for area, isolation (measured as latitudinal differ- ences between sites) adds significant insight into explaining variation in ant community structure.
With little scientific inquiry into the rapidly expanding cityscape, our results show that significant differences exist between microenviron- ments and that rather than addressing urban systems as one large spe- cies poor environment, it may be better addressed as an urban mosaic. Our finding that street medians were the most species rich environment, followed by urban parks, and lastly green rooftops (Fig. 2) is contrary to our prediction that green rooftops, which are presumably most similar to natural environment, would possess the richest communities. The pattern observed in this study may be due in part to the isolation and size variation of the different microenvironments. Green rooftops, being restricted to roof- tops are significantly smaller (mean +/- SE = 743.9 +/- 227 m2) compared to urban parks (mean +/- SE = 1,008,210 +/- 679,707 m2) and street me- dians (mean +/- SE = 1,927 +/- 505 m2). Green rooftops also exist above ground level. This creates a sky island effect in which green rooftops act as isolated islands surrounded by cement and are difficult to reach verti- cally. The isolation of green rooftops may partly explain their low degree of within site similarity in community composition (Table 1). Green rooftop communities also significantly differed from the other microenvironment communities (Fig. 5). One potential explanation is that all rooftops sam- pled were comprised of low vegetation, lacking trees and shrubs making them more exposed to the elements and reducing the vertical complexity of the environment when compared to urban parks and street medians. The community differences observed between green rooftops and other micro- environments were driven largely by Tapinoma sessile and Crematogaster cerasi which each increased in abundance (Table 2). Both of these spe- cies are considered house pests and closely associate with human made structures which may explain their increased abundance on green rooftops (Smith 1928). Green rooftops, may select for species that can both tolerate relatively extreme conditions or species that engage in commensal rela- tionships with humans (Kark et al. 2007, SÆTRE et al. 2012). This pattern
differed from much of the literature which argues that green rooftops may house unique and rare species (Kadas 2006, Madre et al. 2013). Still other studies indicate that no differences exist between green rooftops and the surrounding microenvironments (MacIvor and Lundholm 2011). Ultimately, green rooftops have proven to be very interesting ecologically and the var- ied patterns observed across a multitude of studies warrant further investi- gation into green rooftops and their ecosystems.
Discovering differences between microenvironment communi- ties we wanted to see if area was able to explain the differences observed in species assemblages. First, area was applied in the simplest biogeo- graphic context. The linear regression supports the species area theory. As the area of a site increased, the species richness increased with a z-value of 0.132 (Fig. 3). When assessed individually, only urban street medians maintained a significant relationship (Fig. 4). This finding agrees with the positive z-values present for other invertebrates such as bees and wasps in natural fragments (z = 0.15) (Steffan‐Dewenter 2003). Urban micro- environments, with respect to the species richness area relationship, are similar to naturally fragmented environments (Bolger et al. 2000, Nufio et al. 2009). In addition, our results differ slightly from other urban studies. An investigation of arthropods in urban parks in Tokyo revealed z-values for Diptera and Coleoptera to be 0.235 and 0.222 respectively (Faeth and Kane 1978). Our data, with a z-value of 0.132 is just under most literature values for arthropods in natural fragments. This may be explained by a slight increase in disturbance in urban systems (Dickman 1987, Watling and Donnelly 2006, Savage et al. 2014).
Urban ant communities in this study are different from those found in nearby natural systems. I found 15 fewer total species than were found in surrounding natural savanna sites (Menke and Vachter 2015). However, some species that were most commonly collected in natural en- vironments, Stenamma brevicorne and Brachymyrmex depilis were also present in our study (Menke and Vachter 2015). This connection may indi- cate that natural surrounding savannas are acting as sources for species and are filling the sink microenvironments present within the urban mosaic (Amarasekare and Nisbet 2001). Though there are differences between the natural environment and our microenvironments, urban microenviron- ments also differed from one another.
The differences observed between microenvironment com- munities may be due to a variety of factors. As mentioned area plays a significant role in determining community structure and richness. Howev- er, habitat complexity and disturbance have also been shown to play a key role in determining the ant community structure of urban microenvi- ronments (Savage et al. 2014). Our result of greatest species richness in street medians is contrary to that found by Savage et al. (2014) who found greater species richness in urban parks. This discrepancy may be due to differences in the degree of connectivity present in urban street medians sampled in New York and Chicago as well as the large area of some of our street medians. Connectivity has been shown to play an important role in determining species assemblages for a variety of organisms (Haig et al. 1998, FitzGibbon et al. 2007). With increasing amounts of isolation and decreasing amounts of connectivity we would expect differences in ant communities as colonization and disturbance may have larger impacts on connected habitats (Savage et al. 2014).
Understanding the urban mosaic will provide insight into similari- ties and differences in ecological phenomena that occur in cities compared to natural environments. The connectivity of cities and their occurrence across the globe warrants the study of urban systems (McIntyre 2000, Pickett et al. 2008). Studies have already shown that urban systems select for ant species that tolerate warm and dry conditions (Menke et al. 2011). Some of this selection may be due to the different resources that cities pro- vide to species (White and McDonnell 1988). It has been recently shown that ant species change their behavior and diet to more closely resemble humans within urban systems (Penick et al. 2015). Understanding that ur- ban systems have significant effects on ant community structure helps to strengthen our understanding of urban ecology and investigate further en- vironmental differences that occur within urban systems. Recognizing that urban environments are actually a mosaic of distinct microenvironments is an important step in the field of urban ecology.
Figure 1. A map of the sample sites used in this study; 45 street medians, 12 urban parks, and 8 green rooftops were used. Street median sampling was centered on Ashland Ave.
Figure 2. Sample based species accumulation curves for the three microenvironment types. Shading represent the 95% confidence interval. Species were accumulated significantly faster in parks than green rooftops.
Figure 3. Chao 2 estimated species richness for the three microenvironment types. All three microenvironments signifi- cantly differed from one another based on the lack of overlap of the asymptotic 95% confidence intervals.
Figure 4. A positive linear correlation between Species richness and microenvironment area. (y = 0.6288ln(x) – 0.0505, R2 = 0.308, F1 = 29.54, P < 0.000001)
Figure 5. No significant relationship between area and species richness was observed for green rooftops (A) or urban parks (B). Street medians (C) maintained a positive linear correlation between species richness and the area of the microenvironment (y = 1.5193ln(x) – 5.2543, R2 = 0.5264, F1 = 47.79, P < 0.00001).
Figure 6. A principle coordinate analysis of the community structures of each microenvironment type. The larger symbols represent the average of all sites of that microenvironment type.
Figure 7. The relative abundance of species that comprised at least 1% of the total number of individuals captured. Stars represent significant differences in species abundance and letters indicate which environments differed.
Table 1: Average percent similarity between and within microenvironment types calculated in PERMANOVA
Table 2: SIMPER microenvironments (species ex- plaining 90% of variation)
Acknowledgements
I want to thank my committee for their mentorship and guidance in this research. Without their support and criticism I would not have been able to push myself to a new standard of excellence.
I also wish to thank Cliff Shierk for his extensive help with sam- ple collection over the summer. Without his help I would not have had the excellent data present in this research.
In addition, I wish to acknowledge James Trager for his help with taxonomic identification. He helped to ensure the identifications made in the laboratory were accurate.
Note: Eukaryon is published by students at Lake Forest College, who are solely responsible for its content. The views expressed in Eukaryon do not necessarily reflect those of the College. Articles published within Eukaryon should not be cited in bibliographies. Material contained herein should be treated as personal communication and should be cited as such only with the consent of the author.
References
Agapow, P. M., O. R. Bininda‐Emonds, K. A. Crandall, J. L. Gittleman, G. M. Mace, J. C. Marshall, and A. Purvis. 2004. The impact of species concept on biodiver- sity studies. The Quarterly Review of Biology 79:161-179.
Amarasekare, P., and R. M. Nisbet. 2001. Spatial heterogeneity, source‐sink dynam- ics, and the local coexistence of competing species. The American Naturalist 158:572-584.
Andersen, A. N. 1990. The use of ant communities to evaluate change in Australian terrestrial ecosystems: a review and a recipe. Proceedings of the Ecological Society of Australia 16:347-357.
Andersen, A. N., B. D. Hoffmann, W. J. Müller, and A. D. Griffiths. 2002. Using ants as bioindicators in land management: simplifying assessment of ant community responses. Journal of Applied Ecology 39:8-17.
Andrén, H. 1994. Effects of habitat fragmentation on birds and mammals in land- scapes with different proportions of suitable habitat: a review. Oikos 71:355- 366.
Angold, P., et al. 2006. Biodiversity in urban habitat patches. Science of the Total Environment 360:196-204.
Blair, R. B. 1999. Birds and butterflies along an urban gradient: surrogate taxa for assessing biodiversity? Ecological Applications 9:164-170.
Bolger, D. T., T. A. Scott, and J. T. Rotenberry. 2001. Use of corridor-like landscape structures by bird and small mammal species. Biological Conservation 102:213-224.
Bolger, D. T., A. V. Suarez, K. R. Crooks, S. A. Morrison, and T. J. Case. 2000. Arthro- pods in urban habitat fragments in southern California: area, age, and edge effects. Ecological Applications 10:1230-1248.
Brenneisen, S. 2006. Space for urban wildlife: designing green roofs as habitats in Switzerland. Urban Habitats 4:27-36.
Brown, G. P., B. L. Phillips, J. K. Webb, and R. Shine. 2006. Toad on the road: use of roads as dispersal corridors by cane toads (Bufo marinus) at an invasion front in tropical Australia. Biological Conservation 133:88-94.
Bunn, A. G., D. L. Urban, and T. Keitt. 2000. Landscape connectivity: a conservation application of graph theory. Journal of Environmental Management 59:265- 278.
Carpintero, S., and J. Reyes‐López. 2014. Effect of park age, size, shape and isola- tion on ant assemblages in two cities of Southern Spain. Entomological Sci- ence 17:41-51.
Cervero, R., and K. Kockelman. 1997. Travel demand and the 3Ds: density, diversi- ty, and design. Transportation Research Part D: Transport and Environment 2:199-219.
Ciesin, I. CIAT (2011) Global rural-urban mapping project, Version 1 (GRUMPv1): Urban extents grid. NASA Socioeconomic Data and Applications Center (SE- DAC).
Colwell, R. K. 2005. EstimateS: Statistical estimation of species richness and shared species from samples. Accessed at: http://viceroy.eeb.uconn.edu/estimates/
EstimateSPages/EstSUsersGuide/EstimateSUsersGuide.html
Connell, J. H. 1961. The influence of interspecific competition and other factors on the distribution of the barnacle Chthamalus stellatus. Ecology 42:710-723.
Coovert, G. A. 2005. The ants of Ohio (Hymenoptera: Formicidae). Bulletin of the Ohio Biological Survey 15:1-202.
Coy, M. 2006. Gated communities and urban fragmentation in Latin America: the Bra- zilian experience. GeoJournal 66:121-132.
Cranz, G. 1982. The politics of park design. A history of urban parks in America. Dickman, C. R. 1987. Habitat Fragmentation and Vertebrate Species Richness in an
Urban Environment. Journal of Applied Ecology 24:337-351.
Dunn, R. R., C. R. Parker, and N. J. Sanders. 2007. Temporal patterns of diversity: as- sessing the biotic and abiotic controls on ant assemblages. Biological Journal of the Linnean Society 91:191-201.
Ellis, E. C., and N. Ramankutty. 2008. Putting people in the map: anthropogenic bi- omes of the world. Frontiers in Ecology and the Environment 6:439-447.
Ellison, A. M., N. J. Gotelli, E. J. Farnsworth, and G. D. Alpert. 2012. A field guide to the ants of New England. Yale University Press.
Faeth, S. H., and T. C. Kane. 1978. Urban biogeography. Oecologia 32:127-133.
FitzGibbon, S. I., D. A. Putland, and A. W. Goldizen. 2007. The importance of func- tional connectivity in the conservation of a ground-dwelling mammal in an urban Australian landscape. Landscape Ecology 22:1513-1525.
Foppen, R. P., J. P. Chardon, and W. Liefveld. 2000. Understanding the role of sink patches in source‐sink metapopulations: reed warbler in an agricultural land- scape. Conservation Biology 14:1881-1892.
Gehlhausen, S. M., M. W. Schwartz, and C. K. Augspurger. 2000. Vegetation and microclimatic edge effects in two mixed-mesophytic forest fragments. Plant Ecology 147:21-35.
Gibb, H., and D. F. Hochuli. 2002. Habitat fragmentation in an urban environment: large and small fragments support different arthropod assemblages. Biologi- cal Conservation 106:91-100.
Goldberg, D. E. 1990. Components of resource competition in plant communities. Pages 27-49 in B. J. Grace, and Tilman David, editor. Perspectives on Plant Competition. Academic Press, Inc., San Diego, California.
Haig, S. M., D. W. Mehlman, and L. W. Oring. 1998. Avian movements and wetland connectivity in landscape conservation. Conservation Biology 12:749-758.
Harris, S., and W. Trewhella. 1988. An analysis of some of the factors affecting dis- persal in an urban fox (Vulpes vulpes) population. Journal of Applied Ecology 25:409-422.
Hawley, A. H. 1950. Ecology.
Herkert, J. R. 1994. The effects of habitat fragmentation on midwestern grassland bird communities. Ecological Applications 4:461-471.
Hersteinsson, P., and D. W. Macdonald. 1992. Interspecific competition and the geo- graphical distribution of red and arctic foxes Vulpes vulpes and Alopex lago- pus. Oikos 64:505-515.
Higgins, S. I., and D. M. Richardson. 1999. Predicting plant migration rates in a changing world: the role of long‐distance dispersal. The American Naturalist 153:464-475.
Hitchings, S. P., and T. J. Beebee. 1997. Genetic substructuring as a result of barriers to gene flow in urban Rana temporaria (common frog) populations: implica- tions for biodiversity conservation. Heredity 79:117-127.
Hodgson, J. A., C. D. Thomas, B. A. Wintle, and A. Moilanen. 2009. Climate change, connectivity and conservation decision making: back to basics. Journal of Ap- plied Ecology 46:964-969.
Hogsden, K. L., and T. Hutchinson. 2004. Butterfly assemblages along a human dis- turbance gradient in Ontario, Canada. Canadian Journal of Zoology 82:739- 748.
Holway, D. A., L. Lach, A. V. Suarez, N. D. Tsutsui, and T. J. Case. 2002. The causes and consequences of ant invasions. Annual Review of Ecology and Systemat- ics 33:181-233.
Jackson, L. E. 2003. The relationship of urban design to human health and condition.
Landscape and Urban Planning 64:191-200.
Kadas, G. 2006. Rare invertebrates colonizing green roofs in London. Urban Habitats 4:66-86.
Kark, S., A. Iwaniuk, A. Schalimtzek, and E. Banker. 2007. Living in the city: can anyone become an ‘urban exploiter’? Journal of Biogeography 34:638-651.
King, J. R., A. N. Andersen, and A. D. Cutter. 1998. Ants as bioindicators of habitat disturbance: validation of the functional group model for Australia’s humid tropics. Biodiversity & Conservation 7:1627-1638.
Lenormand, T. 2002. Gene flow and the limits to natural selection. Trends in Ecology & Evolution 17:183-189.
Levine, J. M., P. B. Adler, and S. G. Yelenik. 2004. A meta‐analysis of biotic resis- tance to exotic plant invasions. Ecology Letters 7:975-989.
Maalouf, J.-P., Y. Le Bagousse-Pinguet, L. Marchand, B. Touzard, and R. Michalet. 2012. The interplay of stress and mowing disturbance for the intensity and importance of plant interactions in dry calcareous grasslands. Annals of Bot- any 110:821-828.
MacArthur, R. H., and E. O. Wilson. 1963. An equilibrium theory of insular zoogeog- raphy. Evolution 17:373-387.
MacIvor, J. S., and J. Lundholm. 2011. Insect species composition and diversity on intensive green roofs and adjacent level-ground habitats. Urban Ecosystems 14:225-241.
Madre, F., A. Vergnes, N. Machon, and P. Clergeau. 2013. A comparison of 3 types of green roof as habitats for arthropods. Ecological Engineering 57:109-117.
Marshall, L. G., S. D. Webb, J. J. Sepkoski, and D. M. Raup. 1982. Mammalian evolu- tion and the great American interchange. Science 215:1351-1357.
Martine, G., and A. Marshall. 2007. State of world population 2007: unleashing the potential of urban growth. UNFPA.
Matteson, K. C., J. S. Ascher, and G. A. Langellotto. 2008. Bee richness and abun- dance in New York city urban gardens. Annals of the Entomological Society of America 101:140-150.
McDonnell, M. J., and A. K. Hahs. 2008. The use of gradient analysis studies in ad- vancing our understanding of the ecology of urbanizing landscapes: current status and future directions. Landscape Ecology 23:1143-1155.
McIntyre, N. E. 2000. Ecology of urban arthropods: a review and a call to action. Annals of the Entomological Society of America 93:825-835.
McKinney, M. L. 2002. Urbanization, Biodiversity, and Conservation: The impacts of urbanization on native species are poorly studied, but educating a highly ur- banized human population about these impacts can greatly improve species conservation in all ecosystems. BioScience 52:883-890.
Menke, S. B., B. Guénard, J. O. Sexton, M. D. Weiser, R. R. Dunn, and J. Silverman. 2011. Urban areas may serve as habitat and corridors for dry-adapted, heat tolerant species; an example from ants. Urban Ecosystems 14:135-163.
Menke, S. B., and N. Vachter. 2015. A comparison of the effectiveness of pitfall traps and winkler litter samples for characterization of terrestrial ant (Formicidae) communities in temperate savannas. The Great Lakes Entomologist 47:149- 165.
Montgomery, J. 1998. Making a city: Urbanity, vitality and urban design. Journal of Urban Design 3:93-116.
Morin, P. J. 2009. Community Ecology. John Wiley & Sons.
Murcia, C. 1995. Edge effects in fragmented forests: implications for conservation. Trends in Ecology & Evolution 10:58-62.
Nufio, C. R., J. McClenahan, and E. G. Thurston. 2009. Determining the effects of habitat fragment area on grasshopper species density and richness: a com- parison of proportional and uniform sampling methods. Insect Conservation and Diversity 2:295-304.
Oberndorfer, E., et al. 2007. Green roofs as urban ecosystems: ecological structures, functions, and services. BioScience 57:823-833.
Oke, T. R. 1973. City size and the urban heat island. Atmospheric Environment 7:769- 779.
Palumbi, S. R. 1994. Genetic divergence, reproductive isolation, and marine specia- tion. Annual Review of Ecology and Systematics 25:547-572.
Peacock, J., R. Hine, and J. Pretty. 2007. The mental health benefits of green exer- cise activities and green care. Mind Week Report 2007.
Penick, C. A., A. M. Savage, and R. R. Dunn. 2015. Stable isotopes reveal links between human food inputs and urban ant diets. Proceedings of the Royal Society of London B: Biological Sciences 282: 20142608.
Phillips, B. L., and R. Shine. 2006. An invasive species induces rapid adaptive change in a native predator: cane toads and black snakes in Australia. Proceedings of the Royal Society B: Biological Sciences 273:1545-1550.
Pickett, S. T., M. Cadenasso, J. Grove, C. Nilon, R. Pouyat, W. Zipperer, and R. Cos- tanza. 2008. Urban ecological systems: linking terrestrial ecological, physical, and socioeconomic components of metropolitan areas. Annual Review of Ecological Systems 32:127-157.
Pimentel, D., R. Zuniga, and D. Morrison. 2005. Update on the environmental and economic costs associated with alien-invasive species in the United States. Ecological Economics 52:273-288.
Pinto-Tomás, A. A., M. A. Anderson, G. Suen, D. M. Stevenson, F. S. Chu, W. W. Cle- land, P. J. Weimer, and C. R. Currie. 2009. Symbiotic nitrogen fixation in the fungus gardens of leaf-cutter ants. Science 326:1120-1123.
Poland, T. M., and D. G. McCullough. 2006. Emerald ash borer: invasion of the urban forest and the threat to North America’s ash resource. Journal of Forestry 104:118-124.
Rebele, F. 1994. Urban ecology and special features of urban ecosystems. Global Ecology and Biogeography Letters 4:173-187.
Rehage, J. S., and A. Sih. 2004. Dispersal behavior, boldness, and the link to in- vasiveness: a comparison of four Gambusia species. Biological Invasions 6:379-391.
Rejmánek, M., and D. M. Richardson. 1996. What attributes make some plant species more invasive? Ecology 77:1655-1661.
Říčan, O. i., L. Piálek, R. Zardoya, I. Doadrio, and J. Zrzavý. 2013. Biogeography of the Mesoamerican Cichlidae (Teleostei: Heroini): colonization through the GAARlandia land bridge and early diversification. Journal of Biogeography 40:579-593.
Rice, W. R. 1987. Speciation via habitat specialization: the evolution of reproductive isolation as a correlated character. Evolutionary Ecology 1:301-314.
Saunders, D. A., R. J. Hobbs, and C. R. Margules. 1991. Biological consequences of ecosystem fragmentation: a review. Conservation Biology 5:18-32.
Savage, A. M., B. Hackett, B. Guénard, E. K. Youngsteadt, and R. R. Dunn. 2014. Fine‐scale heterogeneity across Manhattan’s urban habitat mosaic is asso- ciated with variation in ant composition and richness. Insect Conservation and Diversity doi: 10.1111/icad.12098.
Schiller, A., and S. P. Horn. 1997. Wildlife conservation in urban greenways of the mid-southeastern United States. Urban Ecosystems 1:103-116.
Schippers, P., and W. Joenje. 2002. Modelling the effect of fertilizer, mowing, distur- bance and width on the biodiversity of plant communities of field boundaries. Agriculture, Ecosystems & Environment 93:351-365.
Schluter, D. 2009. Evidence for ecological speciation and its alternative. Science 323:737-741.
Sekercioglu, C. H. 2007. Conservation ecology: area trumps mobility in fragment bird extinctions. Current Biology 17:R283-R286.
Simberloff, D., and L. G. Abele. 1982. Refuge design and island biogeographic theory: effects of fragmentation. American Naturalist 120:41-50.
Simms, E. L., and D. L. Taylor. 2002. Partner choice in nitrogen-fixation mutualisms of legumes and rhizobia. Integrative and Comparative Biology 42:369-380.
Simpson, G. G. 1940. Mammals and land bridges. Journal of the Washington Acade- my of Sciences 30:137-163.
Smith, M. R. 1928. The biology of Tapinoma sessile Say, an important house-infesting ant. Annals of the Entomological Society of America 21:307-330.
Smith, R. M., P. H. Warren, K. Thompson, and K. J. Gaston. 2006. Urban domestic gardens (VI): environmental correlates of invertebrate species richness. Bio- diversity & Conservation 15:2415-2438.
Soons, M. B., and J. M. Bullock. 2008. Non‐random seed abscission, long‐distance wind dispersal and plant migration rates. Journal of Ecology 96:581-590.
Steffan‐Dewenter, I. 2003. Importance of habitat area and landscape context for species richness of bees and wasps in fragmented orchard meadows. Con- servation biology 17:1036-1044.
Svirejeva-Hopkins, A., and H.-J. Schellnhuber. 2006. Modelling carbon dynamics from urban land conversion: fundamental model of city in relation to a local carbon cycle. Carbon Balance and Management 1:1-8.
Säumel, I., and I. Kowarik. 2010. Urban rivers as dispersal corridors for primarily wind-dispersed invasive tree species. Landscape and Urban Planning 94:244-249.
Saetre, G. P., Riyahi, S., Aliabadian, M., Hermansen, J. S., Hogner, S., Olsson, U., Gonzales-Rojas, M. F., Saether, S. A., Trier, C. N., and T. O. Elgvin. 2012. Single origin of human commensalism in the house sparrow. Journal of Evo- lutionary Biology 25:788-796.
Tilman, D. 1977. Resource competition between plankton algae: an experimental and theoretical approach. Ecology 58:338-348.
Trakhtenbrot, A., R. Nathan, G. Perry, and D. M. Richardson. 2005. The importance of long‐distance dispersal in biodiversity conservation. Diversity and Distri- butions 11:173-181.
Tsutsui, N. D., A. V. Suarez, D. A. Holway, and T. J. Case. 2000. Reduced genetic variation and the success of an invasive species. Proceedings of the National Academy of Sciences 97:5948-5953.
Tylianakis, J. M., R. K. Didham, J. Bascompte, and D. A. Wardle. 2008. Global change and species interactions in terrestrial ecosystems. Ecology Letters 11:1351- 1363.
Urban, M. C., B. L. Phillips, D. K. Skelly, and R. Shine. 2007. The cane toad’s (Chau- nus [Bufo] marinus) increasing ability to invade Australia is revealed by a dy- namically updated range model. Proceedings of the Royal Society B: Biolog- ical Sciences 274:1413-1419.
US Census Bureau (2010) Census of Population and Housing Demographic Profile Summary File: Technical Documentation.
Von der Lippe, M., and I. Kowarik. 2008. Do cities export biodiversity? Traffic as dis- persal vector across urban–rural gradients. Diversity and Distributions 14:18- 25.
Ward, D. F., R. J. Harris, and M. C. Stanley. 2005. Human-mediated range expan- sion of Argentine ants Linepithema humile (Hymenoptera: Formicidae) in New Zealand. Sociobiology 45:401-407.
Watling, J. I., and M. A. Donnelly. 2006. Fragments as islands: a synthesis of faunal responses to habitat patchiness. Conservation Biology 20:1016-1025.
White, C. S., and M. J. McDonnell. 1988. Nitrogen cycling processes and soil charac- teristics in an urban versus rural forest. Biogeochemistry 5:243-262.
Wichmann, M. C., et al. 2009. Human-mediated dispersal of seeds over long distanc- es. Proceedings of the Royal Society B: Biological Sciences 276:523-532.
Wiens, J. A. 1989. Spatial scaling in ecology. Functional Ecology 3:385-397. Woodroffe, R., and J. R. Ginsberg. 1998. Edge effects and the extinction of popula-
tions inside protected areas. Science 280:2126-2128.
Young, A., T. Boyle, and T. Brown. 1996. The population genetic consequences of habitat fragmentation for plants. Trends in Ecology & Evolution 11:413-418