Characterization of New Familial Mutants of Parkinson’s Disease Protein α-Synuclein Using Yeast Models
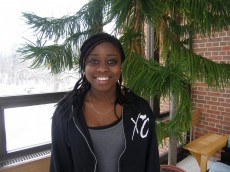
Maiwase Tembo
Department of Biology
Lake Forest College
Lake Forest, IL 60045
Abstract
Parkinson’s disease (PD) is a neurodegenerative disorder associated with the misfolding and aggregation of α-synuclein in midbrain dopaminergic neurons. Familial PD is one of two forms of PD that accounts for 10% of all cases and is the result of genetic mutations. The best-studied gene, α-synuclein, has six known mu- tations. While the first identified mutants (A30P, E46K and A53T) are well studied, little is known about how recently discovered mutants (H50Q, G51D and A53E) create cellular toxicity. For my thesis, I utilized two yeast models to examine the hypothesis that H50Q, G51D, and A53E, each generate toxicity by affecting membrane-association and aggregation properties of α-synuclein. My research has re- vealed that: 1) All mutants alter α-synuclein’s cellular localization. 2) H50Q and A53E increase toxicity, but G51D does not. 3) None of the mutants affect α-synuclein concentration. This thesis provides new insight into familial PD pathogenesis potentially useful for novel therapeutic solutions.
Introduction
Life’s Powerful Workhorses
Life’s astounding diversity lies in the delicate shapes of thou- sands of proteins that serve as functional workhorses in the cells that make up organisms. A slight change in these shapes can cause disease, death or future evolution. Therefore, protein folding must be highly regulated to ensure correct protein function. Nevertheless, errors can occur during pro- tein folding as a result of the environment, cellular stress, and genetic mutations (Shin et al., 2009). An incorrectly shaped protein can be beneficial, neutral or harmful (Figure 1). Beneficial mutations boost the survival of organisms while neutral mutations do not alter protein function. Conversely, harmful mutations lead to proteins that cause a gain or loss of function, and result in disease (Ulloa-Aguirre et al., 2004).
Diseases of Protein Misfolding
Protein misfolding is a natural phenomenon that if not corrected may result in diseases targeting different parts of the body. Although different, the diseases demonstrate the importance of particular proteins in specific cells. For example, a misfolded cystic fibrosis transmembrane conductance regulator (CFTR) causes cystic fibrosis, a disease that results in poor regulation of mucus and digestive enzymes (Chiaw et al, 2009; Figure 2A). On the other hand, misfolded hemoglobin disrupts blood flow to organs and limbs (Stuart and Nagel, 2004; Figure 2A). Other examples are illustrated in Figure 2A (Walker and Levine, 2002; Rubinsztein and Carmicheal, 2003; Westermark, 2005). The effect of misfolded proteins depends on the cell housing the protein and subsequently, the organ where the cell is found (Figure 2B). The most vulnerable organ of such diseases is the brain.
The Brain and Protein Misfolding
A particular class of diseases that affect the brain and are often linked to protein misfolding are called neurodegenerative diseases. They are characterized by progressive neuronal death in specific areas of the CNS. Examples include Alzheimer’s disease (AD), Huntington’s disease (HD), Prion disease, amyotrophic lateral sclerosis (ALS) and Parkinson’s disease (PD). Despite affecting different brain cells, a common characteristic in these diseases is that a protein is misfolding, aggregating and accumulating (Taylor et al., 2002; Waldemar et al., 2007). The proteins come out of solution like yoghurt comes out of milk; these specific proteins are intimately linked to disease pathology. For my thesis, I will focus on PD and its culprit protein, α-synuclein.
Figure 1. Protein folding
Under ideal conditions, RNA is translated into the correct amino acids needed to generate a particular protein with the correct shape. However, a change in one or all the factors influencing protein folding could result in a change in protein shape; genetic factors could alter RNA and ultimately, the amino acids in the protein and the shape the protein forms. On the oth- er hand, the correct amino acids may be present, but cellular stress and environmental factors may modify the protein’s interactions and there- fore, its shape. Misfolded protein may be neutral, beneficial or harmful.
Parkinson’s Disease
PD is the second most common neurodegenerative and movement-based disorder that affects more than four million people (Dorsey et al, 2007; de Silva et al., 2002; Serulle et al., 2006). The average onset of PD is sixty years although risk increases with age (Nass and Przedborski, 2008). The symptoms of PD include bradykinesia, resting tremors and rigidity (Olanow and Tatton, 1999). These symptoms only occur after more than ninety percent of the dopaminergic neurons located in the substantia nigra pars compacta (SNc) are dead. The SNc is part of the basal ganglia, which is the motor circuitry of the brain most affected by PD. The dopaminergic neurons in the SNc are responsible for releasing dopamine (DA), a neurotransmitter that promotes voluntary movement while inhibiting unnecessary involuntary movement (van Raaij et al., 2006; Squire et al., 2012). When the dopaminergic neurons die, DA becomes less available to the basal ganglia; this eventually leads to PD.
Two Forms of PD
PD is classified as either sporadic or familial (Goedert, 2001). Sporadic PD is caused by factors such as the environment and cellular stress while familial PD is caused genetic mutations. Approximately ten percent of PD cases are familial whereas ninety percent occur sporadically. Although the exact causes of sporadic PD are unclear, pesticides, mitochondrial and oxidative stress are possible contributors (Olanow and Tatton, 1999; Dawson and Dawson, 2003). Whether sporadic or familial, PD patients present similar symptoms and pathology. A striking feature of both forms of PD is Lewy bodies; these are vesicular formations containing misfolded, aggregated and accumulated protein (Pollanen et al, 1993; Caughey and Lansbury, 2003). Although the exact role of Lewy bodies in cell death is still unclear, they contain the culprit protein α-synuclein (Da Costa et al., 2000; Tabrizi et al., 2000).
Familial PD has been linked to eleven genes, but only eight have been studied. When these genes are the cause of PD, they contain either autosomal dominant or autosomal recessive mutations. The genes that cause PD through autosomal dominant mutations are SNCA (Polymero- poulus et al., 1997), UCHL1 (Leroy et al., 1998) and LRRK2 (Funayama et al., 2002). The genes that cause PD through autosomal recessive muta- tions are Parkin (Mizuno et al., 2001), PINK1 (Valente et al., 2004), DJ-1 (Bonifati et al., 2003) and ATP13A2 (Najim al-Din et al., 1994). SNCA encodes α-synuclein, the protein that expresses point mutations linked to its misfolding, aggregation and accumulation. SNCA also causes PD through its duplications and triplications (Chartier-Harlan et al., 2004). LRRK2 encodes a protein responsible for intracellular sig- naling and kinase activity (Dachsel et al., 2007). A mutation in the LRRK2 gene increases kinase activity and makes the cells toxic (Greggio et al., 2006). Additionally, LRRK2, Parkin and PINK1 modulate mitochondrial function; when these genes are mutated, they can cause mitochondrial and oxidative stress (Norris and Giasson, 2004). On the other hand, DJ-1 and PINK protect mitochondria during cellular stress, but when mutated they make the mitochondria vulnerable to damage and disease (Deng et al., 2008; Yang et al., 2005) (Figure 3). The genes previously described are all important in PD pathology, however, I focus particularly on familial PD derived from point mutations in the α-synuclein gene, SNCA, and how these mutations affect α-synuclein’s properties.
Figure 2. Diseases of protein misfolding and their cell types
(A) Many parts of the body that can be affected by proteins misfolding as demonstrated in the figure. The examples illustrated are Alzheimer’s dis- ease, Huntington’s disease, Parkinson’s disease, cystic fibrosis, sickle cell anemia, cardiac atrial and aortic medial amyloidosis. In each disease, a particular protein is misfolding Image of body taken from: http://www. buzzle.com/articles/human-body-diagram.html
(B) Different types of cells are affected in the previously mentioned dis- eases. Each cell structure is suited to its functions. Of the cells shown, the brain cell is the most complex in its structure and function. Its struc- ture promotes communication through the use of neurotransmitters. This complex structure and function that make it difficult to regenerate brain cells that may die as a result of protein folding.
α-Synuclein is made of 140 amino acids and the three domains N-, NAC and C- domains (Goedart, 2001;Tan et al., 2004). The N-domain (amino acids 1-60) is amphipathic and promotes lipid binding by forming helices (Chandra et al., 2003). The NAC domain (amino acids 61-95) is hydrophobic and needed for α-synuclein fibrillation. The C-domain is acidic and linked to α-synuclein solubility and flexibility (Bodles et al., 2001; Gias- son et al., 2001; Lücking and Brice, 2001). α-Synuclein is typically located in presynaptic terminals, the cytoplasm and found bound to fatty acids in membranes (Clayton and George, 1998). The protein’s various locations suggest that it plays several roles in the brain.
Despite knowing little about α-synuclein, researchers believe that in a healthy cell α-synuclein regulates dopamine release, aids vesicle transportation and plays a role in endocytosis and endoplasmic reticulum (ER) to Golgi transport (Maroteaux and Scheller, 1991;Jensen et al., 1998; Cabin et al., 2002; Cooper et al., 2006). As such, misfolded α-synucle- in plays several roles that promote cell death (Figure 4). As α-synuclein misfolds and aggregates, it accumulates in the ER/Golgi thereby preventing newly made proteins from being secreted (Cooper et al., 2006; Tardiff, 2013). Moreover, α-synuclein compromises microtubule stability; it encourages tau fibrillation and less tau-microtubule associations, which are necessary for microtubule stability (Lee et al., 2001;Giasson et al., 2003; Sousa et al., 2009). Also, α-synuclein interacts with actin fibers resulting in their destabilization and depolymerization (Sousa et al., 2009; Figure 4). These problems created by α-synuclein exacerbate oxidative stress (already high due to regular oxidation of excess iron/DA) (Lotharius et al, 2002). Oxidative and nitrosative (caused by elevated levels nitric oxide) can further damage the mitochondrial membrane (Tanaka et al., 2001; Pal et al, 2011;Ryan et al, 2013). Although the cellular interactions previously described are linked to PD, the mechanism α-synuclein uses to cause neuronal death is still unclear. A common research area attempts to gain insight into α-synuclein’s induced cell death through studying the effects of α-synuclein genetic mutations, which is what I will discuss next.
α-Synuclein and Familial PD
α-Synuclein has six known mutations located on the N-domain (Figure 5). The three earlier discovered mutations (A53T, A30P and E46K) have been studied in multiple models and their properties have been char- acterized as shown in figure 5. However, the properties of the newer mu- tants (H50Q, G51D and A53E) were discovered and reported in the last three years and their properties are yet to be characterized.
History of Older Familial Mutants
A53T
The first and most well studied α-synuclein point mutation as- sociated with PD was identified in one Greek family and later, three other unrelated Greek families in 1997 (Polymeropoukous et al., 1997). This point mutation exchanges nonpolar alanine for uncharged polar threonine at the 53rd amino acid (Alberts et al. 2009). A53T in vitro and in vivo studies revealed four main findings about its properties: 1) A53T disordered similarly to wildtype (WT) at low concentrations, but formed spherical assemblies at higher concentrations. 2) A53T fibrillated and polymerized faster than WT (Giasson et al., 1998; Conway et al., 2000a). 3) A53T was structurally similar to WT (Jo et al., 2002). 4) A53T and WT both bound membranes in a transgenic mouse model (Sharon et al., 2003). Finally, research conducted in budding yeast models confirmed α-synuclein’s mem- brane binding nature in WT and A53T (at low α-synuclein expression), and added that A53T was not toxic in budding yeast, but was toxic fission yeast, a model in which both WT and A53T formed aggregates (Sharma et., 2006; Brandis et al., 2006).
Figure 3. Genes involved in PD pathology
Several genes are involved in PD. The arrows demonstrate the interactions that occur in the cell. LRRK2 regulates mitochondrial function. When LRRK2 is mutated its function is impaired and cause the mitochondria to alter its func- tion in a way that promotes PD. Additionally, LRRK2 gains a toxic function and promotes kinase dependent cell toxicity. DJ-1, PINK1 and PARKIN form a PDD complex that aids protein degradation when they are not mutated. However, when one of them is mutated, they cannot form this complex and this reduces α-synuclein degradation. α-Synuclein is likely to build up and cause PARKIN to produce substrates toxic to mitochondria. UCHL1 also helps protein degrada- tion and when it is impaired, protein degradation is reduced. Park9 in its regular function protects the cell from manganese toxicity. Therefore impairing Park9 may allow manganese to make the cell toxic.
Figure 4. How α-synuclein affects the cell
α-Synuclein affects the cell in multiple ways. 1) α-Synuclein inhibits the ER/ Golgi pathway by blocking protein secretion (including its own secretion), lead- ing to protein build up in the ER/Golgi 2) α-Synuclein inhibits autophagy and endocytosis, which diminishes the routes that misfolded proteins and other debris may use in order to arrive at the lysosome for degradation. As a re- sult, proteins and other debris accumulate 3) Misfolded α-Synuclein builds up and overwhelms the proteasome so that degradation slows down even further 4) α-Synuclein decreases microtubule stability, which may affect intracellular transport 5) α-Synuclein slows actin polymerization and encourages depolym- erization; this may affect cell structure and compromise the cell’s function.
A30P
A30P was discovered in a British family and is the second most well studied α-synuclein PD mutation. This mutant substitutes nonpolar alanine for nonpolar proline and maintains the overall charge at the 30th amino acid unlike A53T (Kruger et al., 1998). A30P in vitro and in vivo studies resulted in three main findings: 1) A30P did not bind cell membranes (Jensen et al., 1998) 2) A30P was as disordered as WT at low concentration while at higher concentrations, A30P made tiny spherical particles (Conway et al., 1998) 3) A30P formed fibrils at a slow rate (Giasson et al., 1998; Conway et al., 2000a). Interestingly, structural studies revealed that this change in amino acids altered α-synuclein structure (unlike A53T) and prevented it from forming the helices necessary for membrane binding (McLean et al., 2000; Jo et al., 2002). Additionally, an in vitro study, Jensen et al. (1998) tested A30P for vesicle binding and observed no membrane binding. Lastly, budding and fission yeast confirmed that A30P did not bind the membrane, but instead diffused in the cytoplasm (Sharma et al., 2006; Brandis et al., 2006).
E46K
The last of the older familiar mutants was discovered in a Spanish family in 2004. The mutation exchanges negatively charged glutamic acid for positively charged lysine. E46K has been assessed in vitro and in vivo, and these studies revealed three important findings: 1) E46K bound liposomes twice as much as seen in WT, A30P and A53T 2) E46K formed fibrils as fast as A53T in a concentration dependent manner 3) E46K formed aggregates (Choi et al., 2004; Greenbaum et al., 2005). Re- searchers argue that the observed properties are due to E46K’s structure that increased N- and C- domain interactions not seen in A53T and A30P (Bertoncini et al., 2005; Raaij et al., 2006). Furthermore, in vitro studies expressing WT and E46K in neuroblastoma cells revealed that the E46K mutant increased aggregation. Lastly, budding yeast studies confirmed E46K’s lipid binding nature while fission yeast studies confirmed E46K’s ability to form aggregate and added E46K was toxic in fission yeast (Fiske et al., 2011b).
Newer Familial Mutants
H50Q
Since 2013, three new PD linked mutations have been discovered. The first, H50Q, was discovered in an English patient and later in a Canadian patient in 2013 (Proukakis, Houlden & Schapira, 2013; Proukakis et al., 2013; Appel-Cresswell et al, 2013). The H50Q substitutes histidine for glutamine at the 50th amino acid, and is located on the N-domain of α-synuclein
G51D
G51D was discovered in a British patient and is also a mutation located on the N-domain of α-synuclein. The mutation exchanges nonpolar glycine for negatively charged aspartic acid. G51D has also been linked to multiple system atrophy (MSA). Thus, in addition to severe neuronal death in the SNc, the British G51D PD patients had inclusions characteristic of MSA (Kiely et al., 2013). However, other patients with the G51D did not have MSA, but experienced early onset of the disease much like the British patient. The G51D mutant has one of the earliest onsets and fasted progressions of all known familial mutants PD so that patients die within five to seven years of disease onset (Tokutake et al., 2013; Lesage et al, 2013).
A53E
The newest familial mutant was discovered in a Dutch patient and her family in 2014. The patient’s brain pathology was extensive and covered areas of the brain beyond the SNc. The patient’s brain also contained cytoplasmic inclusions and Lewy body inclusions characteristic of MSA and dementia with Lewy bodies (Pasenen et al., 2014). Other than the information learned from the brain pathology of patients with H50Q, G51D and A53E, little is known about the properties of these mutants.
Gap in Knowledge
Whereas the earlier discovered familial mutants (A53T, E46K and A30P) have been well characterized and studied in multiple model organisms, the newer mutants (H50Q, G51D and A53E) have not. Therefore, my thesis will focus on characterizing the properties of the new familial mutants.
Model Organism: Yeast
In order to study the properties of α-synuclein, our lab uses one of the well-known model organisms, yeast. However, there are several models used to study PD such as primates, rats, mice, Drosophila, C. elegans, and cell culture. While primates, rats, and mice are able to model observable PD behaviors seen in PD patients, they are difficult to manipulate genetically, costly, and require ample time (Brownwell et al., 1998; Nass and Prezdborski, 2008). Drosophila and C. elegans model PD successfully because their genes can be easily manipulated, but they require a wide range of assays that make them an undesirable model for our lab (Feany and Bender, 2000; Nass and Prezdborski, 2008; Kuwahara et al., 2006; Nass et al., 2002). Although cell culture is advantageous because it enables the study of living neurons, it is also a very fragile medium. Despite yeast seeming unsuitable, they are useful in the study of PD as demonstrated by several studies (Outiero and Lindquist, 2003; Brandis et al., 2006; Sharma et al., 2006). Consequently, I used two yeast models, budding yeast (Saccharomyces cerevisiae) and fission yeast (Schizosac- charomyces pombe) to study the properties of the newer familial mutants. Our lab and several other labs use yeast to model PD because yeast share many homologous genes with humans. For example, yeast and humans share genes for protein synthesis, folding and degradation. Moreover, budding and fission yeast genomes have been completely sequenced and are readily available as deletion strains making genetic manipulations relatively easy (Outiero and Lindquist, 2003; Willingham et al., 2003; Nass and Prezdborski, 2008). Also, yeasts are cheap, have a short lifespan and reproduce rapidly thus, making them an attractive model for our lab (Nass and Prezdborski, 2008).
The first of my yeast models, is budding yeast, a well-established disease model that has been used to multiple human diseases including neurodegenerative diseases. Examples of neurodegenerative diseases studied in budding yeast include Alzheimer’s (Komano et al., 1998) and Huntington disease (Meriin et al., 2002). To study PD and α-synuclein’s pathological properties, several labs created budding yeast models (Outie- ro and Lindquist, 2003; Zabrocki et al., 2005; Sharma et al., 2006). Yeast does not contain the α-synuclein gene, so researchers use several techniques to express α-synuclein. This gene can either be incorporated into the yeast genome or into a plasmid vector. In their budding yeast model, the Lindquist lab demonstrated that α-synuclein induced toxicity is dose dependent. They established that a single copy of the α-synuclein gene did not cause toxicity while two copies of the gene induced cell death and formed aggregates (Outiero and Lindquist, 2003). Later studies revealed that the observed aggregates were similar to Lewy bodies and vesicles found in human PD brains (Soper et al., 2008).
Our lab has also conducted several studies using yeast. The studies utilized a high copy 2-micron vector to express α-synuclein in budding and fission yeast (Sharma et al., 2006; Brandis et al., 2006). Sharma et al., (2006) developed a budding yeast model to assess α-synuclein toxicity in WT and A53T, and they found none. The lack of toxicity may have as a result of moderated α-synuclein expression levels in their budding yeast model. Next, Brandis et al., (2006) assessed α-synuclein in fission yeast; they discovered that A53T was toxic. Fiske et al., (2011b) then incorporated both budding and fission yeast in their study of E46K; they found E46K was toxic only in fission yeast. Additional studies in our lab assessed the underlying mechanisms of α-synuclein. Fiske et al. (2011a) found that modifying serine phosphorylation altered α-synuclein’s localization. Similarly, senior thesis students evaluated endocytosis (Perez Thesis, 2010; Senagolage Thesis, 2012), and found that several endocytosis genes regulate α-synuclein’s properties.
Other students (Choi Thesis, 2009; Ayala Thesis, 2009) evaluated autophagy, but did not find significant changes in α-synuclein’s proper- ties. Lastly, Natalie Kukulka (Thesis, 2013) and Katrina Campbell (Thesis, 2014) assessed the importance of point mutations; and found that A30P dominates E46K and A53T in combinatory mutants, and that D2, A76, V77, Q79, and E83 are important for the properties of α-synuclein, respectively. Together, these studies demonstrate the strength and usefulness of yeast as a model. Thus I chose to conduct my study in yeast models with the hypothesis and aims to follow.
My Study Hypothesis and Aims
The overall goal of my thesis was to characterize the proper- ties of α-synuclein in the newer familial mutants. I used two yeast models to examine the hypothesis that H50Q, G51D, and A53E, each generate toxicity by altering membrane-association and aggregation properties of synuclein. The hypothesis and predictions are illustrated in figure 6. In order to obtain insight into the characteristics of the individual mutants and assess their less well understood properties, I asked three questions: 1) Where does α-synuclein localize within the each mutant cell? 2) Does α-synuclein make the cells toxic in the individual mutants? 3) Does α-synuclein expression increase in the individual mutants? Each chapter of my thesis addresses one mutant and attempts to answer the previously stated questions using the experimental set-up detailed below.
Experimental Set-up
For my study, I utilized two budding and two fission yeast models. One budding yeast model was low in α-synuclein while the other model was high in α-synuclein (eGFP). For fission yeast models, I used two strains, SP3h+ and TCP1. I chose these models in order to observe different properties of α-synuclein that are strongly linked to PD pathology. The low expression budding yeast model demonstrates α-synuclein’s ability to bind membranes while the two fission yeast strains show α-synuclein’s ability to form aggregates. The high expression budding yeast model also demonstrates both aggregate formation and membrane binding at different time points, thereby showing a combination of the previously stated models’ properties.
To acquire data on the properties of each mutant related to localization, toxicity, and α-synuclein expression, I used three assays: green fluorescent protein (GFP) microscopy, serial dilution spotting and Western analysis. The GFP microscopy allowed α-synuclein visualization within each cell, which enabled me to compare the dominant phenotypes of each mutant at each time point to WT. The spotting assay assessed each mutant’s level of cell growth in comparison to controls: parent vector (pYES2 or pNMT1), GFP alone and the corresponding wild type α-synuclein for each model. Western analysis indicated the level of α-synuclein expressed within the cells. The concentration of α-synuclein in each mutant was compared to the concentration of α-synuclein in WT.
Summary of Main Findings
All mutants alter α-synuclein’s cellular localization. Additionally, H50Q and A53E increase toxicity, but G51D does not. Lastly, none of the mutants affect α-synuclein concentration.
Figure 6. Hypotheses for new familial mutants
(A) In budding yeast, α-synuclein in WT binds to the plasma membrane, but its lo- calization in the newer mutants is yet to be determined. Thus, I predicted that newer mutants could either bind the membrane like WT, diffuse in the cytoplasm or form aggregates. In fission yeast, α-Synuclein in WT forms aggregates whereas its char- acteristics are not known in the newer mutants. Illustrated above are my predictions for the possible phenotypes that each mutant will express.
(B) I expect WT to be less toxic in than each mutant in both budding and fission yeast models. Of the two budding yeast models, I expect that the eGFP (high expression) tagged α-synuclein variants will be more toxic than the GFP tagged. The least growth observed in each budding yeast assay should be the mutant tagged to eGFP Similarly I expect each fission yeast mutant to be more toxic than WT in each strain.
(C) In budding yeast, I expect the each mutant to increase α-synuclein concentration. I expect to observe that the highest α-synuclein in the mutants tagged to eGFP. Like- wise, I expect the mutants in SP3 and TCP1 to increase α-synuclein.
Materials and Methods
All methods described below were adapted from Outiero and Lindquist (2003), Brandis et al. (2006), Sharma et al. (2006) and Fiske et al (2011b).
Creation of α-synuclein Constructs
I began my study by generating three α-synuclein point mutations. I created H50Q G51D and A53E for study in chapter one, two and three, respectively. The methods to be explained were all utilized in my study to assess α-synuclein’s properties. I will begin by describing the yeast strains I utilized.
Yeast Strains
Firstly, the vectors (pYES2.1, pNMT1, GFP, and WT) employed in my experiments were created as described in Sharma et al. (2006) and Fiske et al. (2011). All experiments were conducted using budding and fission yeast. The BY4741 strain containing the pYES2.1 vector was utilized for budding yeast experiments. Each mutant was also tagged with either GFP or eGFP. For fission yeast experiments, the two strains Sp3h+ and TCP1 were used. Both strains contained the pNMT1 vector and were tagged with GFP (Table 1).
Site-directed Mutagenesis
H50Q, G51D and A53E were created using the Invitrogen site-directed mutagenesis kit to create point mutations. For the budding yeast mutants, the pYES2.1 WT α-synuclein template tagged with either GFP or eGFP was mutagenized. For fission yeast, the pNMT1 WT α-synuclein template tagged with GFP was used. Mutagenesis was conducted according to the GENEART Site-Directed Mutagenesis System Protocol specified by Invitrogen Life Technologies. The primers used are shown in table 2. The mutagenesis products were transformed into One Shot Max Efficiency Dh5α-T1 component cells, which were then grown in LB+Ampicillin media. The Dh5α-T1 cells were mini-prepped using a Qiagen Mini-prep Kit to isolate plasmid DNA. The purified DNA was then sent to the University of Chicago DNA Sequencing Facility. Once sequence confirmed, the purified DNA was transformed into yeast as shown in figure 7.
Yeast Expression
The pYES2.1 and pNMT1 vectors were transformed into budding and fission yeast as described by Burke et al. (2000). The budding yeast cells were grown in media lacking the uracil nucleotide (indicated by SC-Ura) as a selection process. Additionally, budding yeast contains a GAL promoter in their vector that controlled their repression and expression of the α-synuclein protein depending on the media used. When grown in SC-Ura-glucose, budding yeast repressed α-synuclein and when grown in SC-Ura+- galactose, budding yeast expressed α-synuclein. The fission yeast cells used were grown in Edinburg Minimal Media (EMM). Similarly to budding yeast, fission yeast either expressed or repressed α-synuclein in a thiamine dependent manner. The yeast expressed a high level of α-synuclein in the absence of thiamine and expressed a low level of α-synuclein (repressed) in the presence of thiamine.
GFP Fluorescent Microscopy
Yeast cells were grown overnight in either 5mL SC-Ura-glucose (budding yeast) or EMM+T (fission yeast) at 30°C and 200rpm. The cells were pelleted at 1500x g for five minutes and washed three times with 5mL of water. A cell density of 2.0x107 cells/mL was transferred to SC-Ura+- galactose for budding yeast and EMM-T for fission yeast. At 12, 24, 36 and 48 hours after the transfer, 1mL of cell culture was removed from the SC-Ura+galactose or EMM-T media and pelleted. Next, 4 microliters of the pelleted product was pipetted onto a glass slide and observed at 1000X using a TE2000-U Nikon fluorescent microscope.
Images were acquired and quantified using Metamorph 4.0 software. The phenotype of each counted cell was classified according to the following criteria:
Statistics:
GFP fluorescent microscopy results were represented in a graph for each α-synuclein variant utilized in my study. Each mutant was graphed alongside WT for each phenotype over the 48-hour time course. T-test analysis was conducted for WT and each mutant at each specific time point and for each phenotype observed. No other statistics were conducted in this study.
Spotting Assay
Yeast cells were cultured in 5mL of SC-Ura+glucose or EMM+T overnight at 30°C and 200 rpm. To collect cells, the yeast were pelleted at 1500 x g for 5 minutes at 4°C. The cells were washed three times with 5mL water, re-suspended in 10mL of water and counted using a hemocytometer to calculate cell density. A volume containing 2.0x106 cells was removed from the 10mL suspension and pelleted. The supernatant was removed and the yeast cells were re-suspended in 1mL to a concentration of 2.0x106 cells/mL. The Cells were then diluted 5 fold in a 96 well microtitier plate and spotted onto SC-Ura+glucose or SC-Ura+galactose for budding yeast and EMM+T or EMM-T for fission yeast. The cells were grown for 48 hours and imaged at 24 and 48 hours using an HP Canoscan scanner and Adobe Photoshop CS3.
Western Blot Assay: α-synuclein Expression
Budding and fission yeast cells were grown overnight in repressive SC-Ura+Glucose or EMM+T respectively. The cells were then pelleted at 1500 x g for 5 minutes at 4°C, washed three times with water, re-sus- pended in water and a cells were counted using a hemocytometer. Yeast cells samples indicative of 2.5x107 cells/mL were washed with washed with100 mM NaN3 and solubilized in electrophoresis sample buffer (ESB) (Burke et al. 2000). The ESB contained of 2% sodium dodecyl sulfate (SDS), 80 mM Tris (pH 6.8), 10% glycerol, 1.5% dithiothreitol, 1 mg/ml bromophenol blue, and a mixture of protease inhibitors and solubilizing agents: 1% Triton-X 100, 1 mM phenylmethylsulfonyl fluoride, 1 mM benzamidine, 1 mM sodium orthovanadate, 0.7 mg/mL pepstatin A, 0.5 mg/mL leupeptin, 10 mg/mL E64, 2mg/mL aprotinin, and 2 mg/mL chymostatin. The cell lysates produced were at 130 volts on a 10-20% Tris-Glycine gel (acquired form Invitrogen) using 1 x SDS running buffer. The size of the protein was determined using SeeBlue ladder (acquired form Invitrogen) as the standard molecular ladder.
The gels were transferred to Polyvinylidene fluoride (PVDF) membranes using the semi-dry transfer technique. The membrane was probed with different antibodies. To detect α-synuclein, either anti-mouse monoclonal anti-V5 (acquired from Invitrogen) or anti-α-synuclein (obtained from Santa Cruz). The anti-α-synuclein was followed by the addition of a secondary antibody. The control used for budding yeast experiments was phosphoglycerokinase (PGK) antibody (obtained from Molecular Probes) while anti-beta-actin (acquired from Abcam) was used for fission yeast.
Table 1. α-Synuclein strains used
The table above describes the different α-synuclein constructs used in the experi- ments conducted. Depicted are the mutants and controls and their respective plas- mids, strains and fluorescent tags.
Table 2. Familial mutant primer design
The primers depicted in the table were designed to create the α-synuclein familial mutants with point mutations matching the desired amino acid change. Each DNA is at the center of each primer.
Figure 7. Creating the familial mutants
The mutants were creating using PCR and an Invitrogen site-directed mutagen- esis kit. WT plasmid (either pYES2.1 or pNMT1) was placed with the appropriate combination of forward and reverse primers, and additional solutions that promoted methylation. Once the WT was methylated, the complimentary forward and reverse primers aligned themselves and recreated a template with mutations prompted by the primers. This template was then recombined to form a plasmid with the mutation. This plasmid was then transformed into E. coli cells that amplified the mutant DNA. Once the plasmid was amplified, it was purified and sequence verified by the University of Chicago DNA sequencing facility. Once the correct sequence was obtained, this plas- mid DNA was transformed into yeast cells such as the ones I analyzed in my study.
CHAPTER 1
CHARACTERIZATION OF α-SYNUCLEIN FAMILIAL MUTANT H50Q α-Synuclein properties in Yeast
To begin my study, I first examined the properties of α-synuclein in budding yeast and then in fission yeast. To determine α-synuclein’s properties in these familial mutants, I assessed the properties of localization, toxicity and expression in WT α-synuclein. The WT variants of α-synuclein utilized in my first chapter (H50Q) were also used in chapter two (G51D) and chapter three (A53E). I will begin my describing my results of H50Q in the low expression budding yeast model.
Low Expression Budding Yeast Model (GFP)
H50Q Binds the Plasma Membrane Like WT
The first experiment I conducted assessed α-synuclein localization. I observed that WT forms aggregates at 12 hours post α-synuclein induction. This phenotype does not persist. At 24 hours WT binds the membrane and continues to until 48 hours (Figure 8A). These results only replicated previous findings from 24 hours onwards; Sharma et al. (2006) did not report aggregates. Similarly, H50Q bound the membrane and ex- pressed this phenotype even earlier than WT. Both WT and H50Q demonstrated cytoplasmic diffusion, while binding the membrane except at 24 hours where WT only bound the membrane with no cytoplasmic diffusion (Figure 8A). Statistical analysis demonstrated that WT and H50Q were not different in how they expressed their phenotypes for α-synuclein except at five points (Figure 8B). H50Q bound the plasma membrane (24 hours, p=<.05), diffused in the cytoplasm (36 hours, p=<.01), and simultaneously bound the membrane and diffused into the cytoplasm more than WT (p=<.01). Furthermore, WT expressed the plasma membrane phenotype/ aggregates phenotype (24 hours, p=<.05) as well as formed more aggre- gates than H50Q (12 hours, p=<.05; Figure 8B).
High Expression Budding Yeast Model (eGFP) H50Q Binds the Plasma Membrane Like WT
As in the low expression budding yeast model, I began my experiments by establishing WT’s phenotype during time course microscopy. Except for 12 hours post α-synuclein induction where WT formed aggre- gates, WT persistently bound the plasma membrane; at 24 and 48 hours this was coupled with cytoplasmic diffusion (Figure 9A). As previously observed in the low expression budding yeast model, H50Q bound the continuously bound the plasma membrane, but at 12 and 24 post induc- tion, H50Q bound the plasma membrane and diffused in the cytoplasm. Statistical analyses indicated that there was no significant difference between H50Q and WT in how they expressed the phenotypes for plasma membrane binding, cytoplasmic diffusion, cytoplasmic diffusion/plasma membrane, plasma membrane aggregates and aggregates (Figure 9B).
Toxicity in Budding Yeast
H50Q is More Toxic than WT in the Low Expression Model
Next, I assessed whether H50Q was toxic in budding yeast. I hypothesized that H50Q would cause toxicity and found that my hypoth- esis was partially supported. In comparison to the three controls, parent plasmid (cells without α-synuclein), GFP alone (also without α-synuclein) and WT-GFP, I found that H50Q-GFP exhibited toxicity (Figure 10A). While WT-eGFP and H50Q-eGFP were both more toxic than the GFP variants and previously described controls, WT-eGFP was more toxic than H50Q-eGFP. The uniform growth on repressive media suggests that the only factor influencing cell death was α-synuclein.
α-Synuclein Expression in Budding Yeast H50Q expression was Identical to WT
Finally, I examined the α-synuclein expression level of H50Q in comparison to WT in my low and high expression budding yeast models. Depicted in figure 8B are three of my attempts. I encountered several prob- lems when acquiring α-synuclein expression data. The first blot in the top panel (trial 1) was not exposed to the visualization solution long enough to observe all bands. The complimentary 48-hour blot was overexposed to the visualization solution and exhibited saturated bands. The second blot (trial 2) shown in top panel was exposed to visualization solution for the correct amount of time, however, I incorrectly loaded the H50Q-eGFP sample for the 48 hour expression. This is shown by the by a higher than expected H50Q-eGFP band. The last trial was evenly loaded and devel- oped correctly, but illustrated curved bands (Figure 8B).
Despite the different problems, the blots consistently demonstrated that there was no difference in α-synuclein expression between WT and H50Q for both low and high expression models at the 24 and 48 hour time points (Figure 8B). Additionally, results illustrated that 48 hours post α-synuclein induction, both WT and H50Q expressed more α-synuclein than observed at 24 hour time point.
SP3 Fission Yeast Strain
H50Q binds the endomembrane more than WT
The next set of experiments conducted for H50Q evaluated the previously described properties of α-synuclein in fission yeast. I began by establishing the phenotypes expressed by WT over 48 hours, and then comparing H50Q to WT. I found that in WT, α-synuclein diffused in the cy- toplasm at 12 hours, but bound the endomembrane from 24 hours onward (Figure 11A). This data did not replicate previous findings that showed that WT SP3 formed aggregates (Brandis et al., 2006; Fiske et al., 2011b). Nevertheless, I compared my WT variant to H50Q. The time course mi- croscopy results revealed that H50Q diffused in the cytoplasm from 12 to 24 hours, but bound the endomembrane from 36 hours onward. No statistical difference existed between WT and H50Q for all the phenotypes expressed except for endomembrane binding at 36 hours; H50Q bound the endomembrane significantly more than WT (p=<.05) (Figure 12B).
TCP1 Fission Yeast Strain
H50Q Binds the Endomembrane More than WT
Similar to SP3 fission yeast experiments, I began by assessing WT α-synuclein properties in TCP1. I observed that WT diffused in the cytoplasm from 12 to 24 hours, but formed aggregates at 36 and 48 hours (Figure 12A). The persisting aggregates observed in WT replicated the findings of Brandis et al. (2006). I then compared WT to H50Q; H50Q dif- fused in the cytoplasm in the first 25 hours, but bound the endomembrane from 36 hours onward. The statistics conducted revealed that there was no statistically significant difference between WT and H50Q in the phenotypes they expressed (Figure 12B).
Toxicity in Fission Yeast
H50Q is Less Toxic than WT
My next goal was to assess how H50Q affected cellular toxicity in fission yeast. I utilized PP, GFP, and WT as controls for each H50Q mu- tant. I observed that under low α-synuclein expression conditions (control), even the controls were slightly toxic for both strains (Figure 13A). This toxicity replicated previous findings in our lab (Sharma et al., 2006). Under high α-synuclein expression conditions, WT was toxic, and grew equally to H50Q. Since H50Q did not grow less than WT in SP3, I concluded that it was not toxic in SP3. Surprisingly, the data suggested that WT was more toxic than H50Q in TCP1. (Figure 13B).
α-Synuclein Expression in Fission Yeast
Expression Assay Inconclusive
The last assessment I performed of α-synuclein properties in H50Q fission yeast mutants attempted to evaluate the level of α-synuclein expression. Each trial failed to exhibit any α-synuclein or control bands. Therefore, protein expression could not be interpreted. Exhibited in Figure 13B are two representations of my attempts using different antibodies.
Figure 8. Microscopy and quantification of H50Q in the low expression model
(A) The microscopy images shown are of the dominant phenotype observed for WT and H50Q over 48 hours. The images were captured at 12, 24, 36, and 48 hours post induction with SC-Ura galactose (n=5).
(B) The time course quantification for each phenotype exhibited by WT and H50Q is represented in the bar graphs (n=5). The error bars represent the standard deviation based on the mean. A * above bars represents a significant difference at the p<.05 while ** represents a significant difference at the p<.01).
Figure 9. Microscopy and quantification of H50Q in the high expression model
(A) The microscopy images shown are of the dominant phenotype observed for WT and H50Q over 48 hours. The images were captured at 12, 24, 36, and 48 hours post induction with SC-Ura galactose (n=5).
(B) The time course quantification for each phenotype exhibited by WT and H50Q is represented in the bar graphs (n=5). The error bars represent the standard deviation based on the mean. A * above bars represents a significant difference at the p<.05) while ** represents a significant difference at the p<.01).
Figure 10. Toxicity and protein expression of H50Q in budding yeast
(A) Five-fold serial dilution assay of yeast expressing PP, GFP, WT-GFP, H50Q-GFP, WT-eGFP and H50Q-eGFP α-synuclein spotted onto repressive media (SC-Ura glu- cose) and inductive media (SC-Ura galactose) (n=3).
(B) Western blot trials at 24 and 48 hours of α-synuclein induction for WT-GFP, H50Q- GFP, WT-eGFP, and H50Q-eGFP. The α-synuclein expression was observed using anti- α-synuclein and an anti-PGK loading control.
Figure 11. Microscopy and quantification of H50Q in SP3 fission yeast
(A) The microscopy images shown are of the dominant phenotype observed for WT and H50Q over 48 hours. The images were captured at 12, 24, 36, and 48 hours post induction with EMM-T (n=5).
(B) The time course quantification for each phenotype exhibited by WT and H50Q is represented in the bar graphs (n=5). The error bars represent the standard deviation based on the mean. A * above bars represents a significant difference at the p<.05) while ** represents a significant difference at the p<.01).
CHAPTER 2
CHARACTERIZATION OF α-SYNUCLEIN FAMILIAL MUTANT G51D
Low Expression Budding Yeast Model (GFP)
My experimental goals for G51D were the same as described
for H50Q in chapter one. I utilized the same WT variants and controls to compare how G51D affects α-synuclein’s properties in budding and fission yeast. I will first describe my findings in budding yeast.
G51D Binds the Plasma Membrane Less than WT
First, I will summarize WT’s properties as described in chapter one. WT formed aggregates at 12 hours post α-synuclein induction and persistently bound the membrane beyond 12 hours (Figure 14A). In con- trast, G51D predominantly diffused in the cytoplasm and bound the plasma membrane less than WT. While there was no statistical difference in plas- ma membrane binding between WT and G51D at 12 and 48 hours, WT bound the plasma membrane more significantly than G51D at 24 (p=<.01) and 36 hours (p=<.01). Another significant statistical difference occurred in the level of cytoplasmic diffusion observed in WT and G51D at 36 hours; G51D (p=<.05) diffused in the cytoplasm more than WT (Figure 14B). Oth- er significant differences observed included that: WT expressed the plas- ma membrane/aggregate phenotype at 12 (p=<.01) and 36 hours (p=<.05) and that WT aggregated more than G51D at 12 (p=<.05) and 24 hours (p=<.01; Figure 14A).
High Expression Budding Yeast Model (eGFP) G51D Binds the Plasma Membrane less than WT
Subsequently, I evaluated whether G51D also altered α-synu- clein’s properties in the high expression budding yeast model. Utilizing WT-eGFP (formed aggregates at 12 hours and bound the membrane persistently-described in chapter one) as a control, I observed that G51D bound the plasma membrane less than WT as seen in the low expres- sion budding yeast model (Figure 15A). Additionally, statistical analyses confirmed this finding of WT binding the membrane less than G51D at 12 (p=<.05), 24 (p=<.01) and 36 hours (p=<.01) (Figure 15B). On the other hand, G51D diffused in the cytoplasm significantly more than WT at 12 (p=<.01), 24 (p=<.01), 36 (p=<.01) and 48 hours (p=<.01). Moreover, WT and G51D were statistically different in their levels of cytoplasmic diffu- sion/plasma membrane binding; WT expressed this phenotype more than G51D at 12 (p=<.05) and 24 hours (p=<.01). Lastly, WT aggregated more than G51D at 12 hours (p=<.05; Figure 15B)
Toxicity in Budding Yeast G51D is less toxic than WT
Next, I assessed G51D’s toxicity utilizing PP, GFP and WT as controls. I found that G51D-GFP was not toxic; WT and G51D cells grew equally (Figure 16A). Surprisingly, G51D-eGFP was less toxic than WT; while WT-eGFP exhibited cellular toxicity, G51D-eGFP was less toxic. The uniform growth of all yeast cells suggested that α-synuclein was the only factor influencing cell growth.
α-Synuclein Expression in Budding Yeast G51D Expression was identical to WT
Lastly, I evaluated α-synuclein concentration in G51D for both budding yeast models as previously done for H50Q in chapter one. Illus- trated in figure 16B is a representation of my efforts. These Westerns were conducted at the same time as the H50Q Westerns, thus the same issues described in chapter one affected them. Nevertheless, the data were in- terpretable and suggested that G51D and WT expressed equal levels of α-synuclein at 24 and 48 hours post α-synuclein induction. Additionally, the data consistently demonstrated that both WT and G51D exhibited a higher α-synuclein concentration at 48 hours than observed at 24 hours in both yeast models.
SP3 Fission Yeast Strain
G51D Binds the Endomembrane Less than WT
Thereafter, I evaluated the properties of α-synuclein in fission yeast. The first strain I will discuss is SP3. As mentioned previously, WT first diffused in the cytoplasm at 12 hours and then bound the endomem- brane for the rest of the time course. In stark contrast, G51D diffused in the cytoplasm from 12 to 36 hours and only bound the endomembrane at 48 hours. Despite the contrasting phenotypes shown in figure 17A, the only significant statistical difference observed was at 36 hours where G51D dif- fused in the cytoplasm more than WT (p=.01; Figure 17A).
TCP1 Fission Yeast Strain G51D Aggregates Less than WT
Then, I analyzed G51D in TCP1 as conducted for SP3. I com- pared the properties of G51D to WT (described in chapter one). I found that similar to SP3, G51D diffused in the cytoplasm from 12 to 36 hours, and then bound the plasma membrane and endomembrane at 48 hours (Figure 18A). I conducted statistical analyses that revealed that there was no significant difference in aggregate formation in WT and G51D. However, the results showed significant differences as follows: WT bound the endomem- brane and diffused in the cytoplasm significantly more than G51D at 12 hours (p=.05), G51D diffused in the cytoplasm significantly more than WT at 24 (p=.05) and 48 hours (p=.05), and G51D bound the endomembrane significantly more than WT at 48 hours (p=.01; Figure 18B).
Toxicity in Fission Yeast G51D is less toxic than WT
The goal of my next experiment was to assess how G51D affect- ed cellular toxicity in fission yeast. I used the same controls as described in chapter one to assess α-synuclein. On low α-synuclein expression media, the yeast grew equally, however under high α-synuclein expression con- ditions, WT was toxic. G51D was still less toxic than WT as seen in SP3 (Figure 19A).
α-Synuclein Expression in Fission Yeast Expression Assay Inconclusive
Finally, the Western blot comparing G51D to WT in both fission yeast strains cannot be interpreted due to poor membrane development. Figure 19B is a representation of my efforts.
CHAPTER 3
CHARACTERIZATION OF α-SYNUCLEIN FAMILIAL MUTANT A53E
Low Expressing Budding Yeast Model (GFP) A53E Binds the Plasma Membrane Less than WT
For the final mutant, A53E, I conducted the same experiments described in chapters one and two. Therefore, I used the same controls. In my time-course microscopy, I found that A53E primarily bound the en- domembrane, while it also diffused in the cytoplasm from 12 to 48 hours (Figure 20A). No significant differences were observed between WT and A53E except at 24 and 36 hours where WT expressed the plasma mem- brane/aggregates more than A53E (p=.05), and 24 hours when WT aggre- gated more than A53E at 24 hours (p=.05; Figure 20B).
High Expressing Budding Yeast Model (eGFP)
A53E Binds the Membrane less than WT
Next, I assessed α-synuclein’s properties in A53E’s high expres- sion model. WT (as described in chapter 1 and 2) formed aggregates at 12 hours and bound the plasma membrane at 24, 36 and 48 hours (Figure 21A). However, A53E diffused in the cytoplasm at 12 hours, both bound the plasma membrane and diffused in the cytoplasm at 24 hours. Then, A53E bound the membrane at 36 hours before it diffused into the cytoplasm at 48 hours (Figure 21A). I conducted statistical analyses to compare A53E and WT on each dominant phenotype observed. No significant differences were observed except at 24 hours when WT bound the membrane more than A53E (p=.010; Figure 21B).
Toxicity in Budding Yeast A53E and WT Grow Equally
Subsequently, I investigated how A53E causes toxicity. A53E grew equally to WT in both the high and low expression models. The simi- lar growth patterns observed for all samples on the repressive media sug- gests that only α-synuclein is affecting cell growth (Figure 22A).
α-Synuclein Expression in Budding Yeast A53E Does Not Alter α-Synuclein Expression
The last assay conducted for the budding yeast models as- sessed the concentration of α-synuclein in A53E. The Western blots in figure 22B are representative of my attempts; the data reveals that of α-sy- nuclein concentration did not increase in both models at 24 and 48 hours of α-synuclein induction. Additionally, the Western blot demonstrates that more α-synuclein was expressed at 48 hours than at 24 hours.
SP3 Fission Yeast Strain
A53E Forms Less Aggregates than WT
The last set of experiments evaluated A53E in fission yeast. In SP3. A53E diffused in the cytoplasm at 12 hours, formed aggregates at 24 hours, bound the plasma membrane and the endomembrane at 36 hours, and bound only the endomembrane at 48 hours (Figure 22A). I compared the phenotypes expressed in A53E to WT and found that there was no significant difference between A53E and WT for each of the phenotypes shown (Figure 22B).
TCP1 Fission Yeast Strain A53E Aggregates Less than WT
In this TCP1 fission yeast model (also described in chapter 1 and 2), WT α-synuclein diffuses in the cytoplasm at 12 hours, binds the endomembrane at 24 hours, simultaneously binds the plasma membrane and the endomembrane at 36 hours, and only binds the endomembrane at 48 hours (Figure 23A and 23B). A53E diffuses in the cytoplasm at 12 hours, binds the endomembrane at 24 hours, diffuses in the cytoplasm at 36 hours, and demonstrates a combination of plasma membrane and en- domembrane binding at 48 hours. WT and A53E did not differ significantly in any phenotype expressed at 12, 24, 36, and 48 hours .
Toxicity in Fission Yeast A53E is Toxic in SP3
Next, I assessed cellular toxicity in SP3. Cell spotting was not uniform across all samples on the repressive media plates (Figure 25A). Particularly, WT-GFP in the TCP1 strain grew significantly less on the low expression plates. Thus, I could not conclude. However, A53E in SP3 grew equally to the controls on the low expression media. As a result I evaluated the toxicity shown in SP3 and found that there was no difference in growth between A53E and WT.
α-Synuclein Expression in Fission Yeast Expression Assay Inconclusive
Lastly, as in chapter 1 and chapter 2, I performed a Western blot analysis of A53E to compare it to WT and determine the level of α-synucle- in it expressed. The Western developed poorly. Therefore, protein expres- sion could not be assessed. Illustrated in Figure 25B are representative blots of my attempts.
Discussion
A combination of several factors, including sporadic and familial, can be attributed to the onset of PD. Whilst 90% of all PD cases arise sporadically, 10% originate from mutations in PD associated genes. Six point mutations in the α-synuclein gene account for a proportion of 10% of familial PD and are identified as: A53T, E46K, A30P, H50Q, G51D and A53E. Unlike the older mutants (A30P, E46K and A53T), the newer mu- tants (H50Q, G51D and A53E) have not been well characterized in multi- ple model organisms. Thus, the goal of my thesis was to create and then characterize α-synuclein’s localization, its induced toxicity and expression in each H50Q, G51D, and A53E. Using budding and fission yeast, I found: 1) all three mutations alter α-synuclein’s localization 2) H50Q and A53E are toxic while G51D is not 3) None of the mutants alter α-synuclein expres- sion.
All Three Mutants Alter α-Synuclein’s Localization
My hypothesis was that all mutations would alter α-synuclein’slocalization in budding and fission yeast. This was supported by the data; I found that each mutation within each model exhibited altered α-synu- clein’s properties in distinct ways during the 48 hour time course. H50Q and A53E altered α-synuclein’s localization in all models by decreasing plasma membrane or endomembrane binding (fission yeast). Although both mutants decreased overall membrane binding in comparison to WT in budding yeast, the results showed that both mutants have a high affinity for lipids as they bound the endomembrane in fission yeast. This study is one of the first to demonstrate that both H50Q and A53E bind the plasma membrane (as well as the endomembrane in fission yeast models) in vivo. My data agrees with the in vitro assays that demonstrated that H50Q col- lects in micelles; since micelles also contain lipids, this explains why I ob- served H50Q binding lipids across models (Khalaf et al., 2014). Likewise, a budding yeast study also confirmed H50Q’s affinity for lipids, since H50Q binds the plasma membrane as was seen in my study (Fares et al., 2014). Although no such studies of A53E have been conducted yet, A53E shares the same location and switches from a nonpolar to a polar amino acid as A53T. Therefore, A53E may share properties found near the point mutation that promote membrane binding (Sharon et al., 2003; Sharma et al., 2006; Brandis et al., 2006).
H50Q and A53E lie in the N-domain (amino acid 1-57) that is responsible for the membrane association properties of α-synuclein (Soper et al., 2008). This may explain why they bind membranes. Surprisingly, G51D also lies in the N domain, but diffuses in the cytoplasm across all models utilized in my study. This suggests that G51D lies in the part of the N do- main crucial for membrane binding. Further studies explain this dominant phenotype to be a result of G51D disrupting the α-synuclein helix neces- sary for membrane binding. Another study confirmed my findings in their budding yeast model (Fares et al., 2014). This observed phenotype is simi- lar to A30P (Jensen et al., 1998; Sharma et al., 2006; Brandis et al., 2006). In addition to membrane binding, I expected the mutants to form more aggregates than WT in both budding and fission yeast models, but they did not. Firstly, G51D hardly formed aggregates while H50Q and A53E did, but not significantly more than WT. Conversely, a budding yeast study re- vealed greater aggregate formation in H50Q than in WT models (Fares et al., 2014). Additional in vitro studies of α-synuclein also supported higher aggregation in H50Q than WT. (Rutherford et al., 2014; Khalaf et al., 2014; Ghosh et al., 2013; Porcari et al., 2015). Furthermore, NMR spectroscopy supported H50Q’s role in α-synuclein aggregation by showing that substi- tuting glutamine for positively charged amino acid (arginine) suppressed its aggregation (Chi et al., 2014). The difference between my findings and literature may be explained by differing α-synuclein concentrations; my model systems may not express as much α-synuclein as Fares et al. (2014)’s yeast model.
Whereas H50Q has been evaluated for aggregation, A53E has not. Never- theless, A53T forms minimal aggregates and may explain why A53E does as well (Sharma et al., 2006). Since no studies assessing A53E’s aggrega- tion, I compared it to A53T, which fibrillates and aggregates faster than WT (Conway et al., 1998; Giasson et al., 1998; Conway et al., 2000a; Sharma et., 2006; Brandis et al., 2006). Glutamic acid did not increase aggregation in my model, which may suggest that A53E alters α-synuclein differently from A53T or that A53E suppresses aggregate formation. Alternatively, the α-synuclein concentration may not have been high enough to cause aggre- gation in my models.
Moreover, other studies also demonstrated that G51D rarely formed ag- gregates. In vitro studies illustrated that G51D exhibited a low level of ag- gregation through kinetic studies (Lesage et al., 2013). This was also con- firmed by another study that utilized budding yeast studies and revealed that G51D impeded aggregate formation (Rutherford et al., 2014). G51D mimics the properties of A30P by diffusing and impeding aggregation (Jen- sen et al.; 1998, McLean et al., 2000; Jo et al., 2002; Brandis et al., 2006). Therefore, G51D may cause a conformational change in α-synuclein di- rectly linked to membrane binding. Additionally, data suggests that G51D is a crucial player in the N-domain linked lipid loving nature of α-synuclein.
Two Mutants are Toxic While One is Not
My second hypothesis that all mutants were toxic was partially supported. I found that H50Q and A53E are toxic, but that G51D was not. H50Q was more toxic than WT across all mutants. Just as I demonstrated in my data, in vitro studies confirmed H50Q’s toxicity (Rutherford et al., 2014). Furthermore, a recent budding yeast study also demonstrated that H50Q inhibits cell growth (Fares et al., 2014). Also consistent with my re- sults, other studies demonstrated cell death in hippocampal cells (Khalaf et al., 2014). On the other hand, my data demonstrated that A53E was toxic only in fission yeast. My findings were similar to previous studies that revealed that A53T was toxic in fission but not in budding yeast (Bran- dis et al., 2006; Fiske, et al, 2011). The selective toxicity demonstrated by A53E maybe explained by perturbed ER-Golgi trafficking since A53E demonstrated some level of endomembrane binding. The increase in en- domembrane binding may represent disturbances in ER-Golgi trafficking much like seen when α-synuclein was overexpressed in the budding yeast model (Cooper et al., 2006). All these studies suggest that histidine and alanine play protective roles by preventing cell death.
G51D did not bind the membrane or form aggregates like H50Q and A53E. Since membrane binding and aggregate formation have been linked to cell death (Sharma et al., 2006; Brandis et al., 2006), G51D must cause neuronal death using a different mechanism than H50Q and A53E A recent study in a budding yeast model supported my findings that G51D is not toxic (Fares et al., 2014). Surprisingly, my data added that G51D grew even better than WT. This contrasts in vitro studies that determined that G51D caused toxicity in mammalian cells (Fares et al., 2014; Rutherford et al., 2014). Consequently, the yeast cells must also have underlying mech- anisms that prevent cell death. These results demonstrate the importance of utilizing different models to study α-synuclein.
Multiple Avenues for α-Synuclein Associated Toxicity
α-Synuclein generates toxicity through multiple mechanisms. Toxicity can occur through its aggregation, accumulation, impaired deg- radation, membrane interactions and oligomer formation (Cooper et al., 2006; van Rooijen et al., 2010; Oueberi et al., 2013; Stöckl et al., 2013; Hähl et al., 2014; Lorenzen et al., 2014). I focus on the last two and how they relate to my study. During regular function, α-synuclein binds mem- branes without inducing toxicity. However, in PD, toxicity occurs because the concentration and effects of α-synuclein at the membrane change (van Rooijen et al., 2010). First, α-synuclein increases so that it disrupts vesi- cles traveling to the membrane and prevents membrane regeneration. This destabilizes the membrane and promotes membrane solute permeability (van Rooijen et al., 2010). These changes alter cellular process such as mitochondrial activity; for example increased calcium ions alter mitochon- drial membrane permeability (Luth et al., 2013). Additional changes include multiple pore formation, decreased membrane thickness and inhibited tight packing of the lipid bilayer, which exacerbate membrane leakage (Hähl et al., 2014; Stöckl et al., 2013).
In my study, H50Q and A53E bound membranes and were more toxic than G51D, which rarely bound membranes. Thus, H50Q and A53E may have caused toxicity by altering the properties of the membrane as previously described while G51D’s lack of toxicity may be explained by its inability to alter the membrane. Noting that H50Q and A53E bound mem- branes less than WT, they must have achieved toxicity using additional mechanisms. Perhaps, the toxicity is related to H50Q and A53E’s slight dif- fusion in the cytoplasm. However, H50Q and A53E were not more diffused in the cytoplasm than G51D, therefore the mechanisms utilized to induce toxicity may relate to the form of α-synuclein present in the cells. α-Synu- clein is present as monomers or oligomers, which are both important in α-synuclein’s functions, as well as PD pathology (Burré et al., 2014). Yet, the oligomers have been linked to greater toxicity (Conway et al., 2000b; Li et al., 2001). A high concentration of oligomers impairs membrane function, SNARE complex formation, vesicle fusion, microtubule transport, mito- chondria, proteasomes and lysosomes (Burré et al., 2014; Luth et al. 2014; Smith et al., 2005). Consequently, H50Q and A53E may contain a high lev- el of soluble oligomers that impair cellular function as previously described. Then, G51D may contain more monomers than oligomers so that it does not impair cellular function to the same extent as H50Q and A53E. Since G51D is toxic in PD, it is inducing toxicity differently from H50Q and A53E. Perhaps, G51D’s toxicity affects other aspects of the cell like the level of hydrogen peroxide and cell stress, which have also been linked to toxicity (Wang et al., 2011). Alternatively, G51D’s toxicity may be intimately linked to its structure.
Amino Acid Substitution Drives Toxicity Through Altering Structure
The familial mutants causing more aggressive and earlier onset PD suggest that the some amino acids are key in maintaining the proper- ties of α-synuclein that prevent its toxicity. This toxicity may occur through changes in membrane interactions; membrane binding occurs due to α-synuclein’s positive core being attracted to the negatively charged hy- drophobic heads in the lipid bilayer (Stöckl et al., 2013). In H50Q, A53E, and G51D, a charge change occurs and may be responsible for altering α-synuclein’s structure and thus, its interaction with the membrane. To bind the membrane, α-synuclein first folds into α-helices (Hähl et al., 2014). Therefore, the reduced membrane binding in the three mutants suggests that they decrease α-helix formation. Instead, the mutants may fold into β-sheets, which have been linked to high levels of toxicity in mammalian cells (Cremades et al., 2012).
None of the Mutants Enhance α-Synuclein Expression
My hypothesis that all mutants would express more α-synuclein than WT was not supported. My evaluation of protein expression was hin- dered in two of my four models. All three mutants expressed similar levels of α-synuclein to that of WT, and their high expression models produced more α-synuclein than the low expression models. Therefore, H50Q, G51D and A53E do not enhance α-synuclein expression in budding yeast. Like- wise, H50Q and G51D did not produce more α-synuclein than WT in neuro- nal cells (Rutherford et al., 2014). Nevertheless, in vitro studies contrasted my findings by revealing that H50Q and G51D increased α-synuclein ex- pression (Fares et al., 2014; Khalaf et al., 2014). Similarly, mammalian and neuronal cells enhanced secreted and nuclear levels of α-synuclein (Fares et al., 2014). Thus, G51D may alter α-synuclein transport, its degradation and cause its accumulation in a way that kills mammalian cells, but triggers compensatory mechanisms in budding yeast. In order to determine if the mutants do not increase α-synuclein, these experiments need to be repeated.
On the other hand, A53E has no documented studies evaluating its protein expression. Expecting A53E to behave like A53T, I predicted that A53E would enhance α-synuclein expression, but it did not (Brandis et al., 2006; Kukulka Thesis, 2013). Therefore, glutamate may not alter the structure of α-synuclein in a way that promotes α-synuclein expression in budding yeast. Alternatively, it may induce expression in other models like H50Q and G51D. Furthermore, previous studies showed heightened A53T expression in fission yeast, but not in budding yeast (Brandis et al., 2006; Kukulka Thesis, 2013). Since my fission yeast Western blots were unsuc- cessful, I was unable to support or refute my hypothesis that A53E, H50Q, and G51D increase α-synuclein expression.
All α-Synuclein Mutants Exhibit Unique Profiles
A53T, A30P, and E46K lie in the N domain that is known to promote lipid binding (Chandra et al., 2003). A53T and E46K bind lipids while A30P does not (Sharon et al., 2003, Fiske et al., 2011; Jensen et al., 1998). Moreover, A53T and E46K form aggregates while A30P does not (Sha- ron et al., 2003, Sharma et al., 2006; Brandis et al., 2006; Fiske et al., 2011; Jensen et al., 1998). A53T and E46K are toxic in fission yeast, but not A30P (Brandis et al., 2006; Fiske et al., 2011). However, only A53T increased α-synuclein .
The newer mutants exhibit a combination of the properties ob- served in the older mutants. H50Q most behaves like E46K, G51D most behaves like A30P, and A53E most behaves like A53T. Therefore, H50Q and A53E bind membranes and form aggregates whereas G51D diffuses in the cytoplasm. However, the H50Q and A53E mutants did not demon- strate fission yeast specific toxicity, yet G51D grew as well as WT (as seen in A30P) (Jensen et al., 1998). Lastly, all three mutants did not enhance α-synuclein expression in budding yeast, and due to unsuccessful West- ern blot development, I could not determine if A53E accumulates in fission yeast like A53T.
Conclusion
My Findings
My thesis has provided some understanding into the properties of the three newer α-synuclein mutants. I successfully characterized α-sy- nuclein H50Q, G51D, and A53E in budding and fission yeast models. Each mutant altered α-synuclein localization, H50Q and A53E induced toxicity, and none of the mutants enhanced α-synuclein expression.
α-Synuclein: Amino Acid Importance
Given that protein shape determines protein function, important questions about PD focus on the shape of α-synuclein that induces toxic- ity and changes that alter its shape. In familial PD, genetic changes are responsible for amino acid substitutions that eventually lead to PD. Amino acids are key determinants of protein shape due to their individual proper- ties that promote specific interactions within proteins. For example, amino acids 1-57 create the N-domain necessary for α-synuclein’s lipid binding nature (Bodles et al., 2001; Giasson et al., 2001; Lücking and Brice, 2001). This region houses all six known SNCA mutations and demonstrates the importance not only of the type of amino acid, but also the location of the amino acid within the whole protein. Therefore, characterizing each mutant is essential in understanding α-synuclein.
Already, A53T, A30P and E46K have been well-characterized not only as individual mutants, but also as double and triple mutants. Thus, we now know that A30P dominates the observed phenotype in combinatory mu- tants, and in doing so, suggests that some locations on the protein are more important than others for α-synuclein localization, toxicity, and ex- pression (Brandis et al., 2006; Kukulka Thesis, 2013). Additionally, other amino acids may play important roles in posttranslational modification. For example, S87 and S129 are involved in phosphorylation (Fujiwara et al., 2002). K96 and K102 are involved in sumoylation (Wilkinson et al., 2003). Y39, Y125, Y133, and Y136 are nitration sites (Clayton & George, 1998). More recently, a study identified additional sites important for membrane binding (V15, V26, Y38, E57, E62 N65, E104, E105, and D115 (Tsigelny, 2015). Therefore, characterizing each mutant is important because it can provide insight not only into the amino acid change that occurred, but also the potential posttranslational modifications and underlying mechanisms that prevent or promote α-synuclein induced toxicity.
Future Studies of Newer Familial Mutants
Although my thesis answers some questions, it also raises several unanswered questions: 1) How do H50Q, G51D, and A53E change the properties of α-synuclein? 2) Are the underlying mechanisms that cause PD the same in the H50Q, G51D and A53E? 3) How do these mechanisms compare to A53T, A30P, and E46K? 4) Is cytoplasmic diffusion protective or is it a result of an underlying protective mechanism that prevent toxicity in yeast?
Firstly, I would focus on improving my Western blots. I conduct- ed successful budding yeast Westerns, but not fission yeast. Successful Western techniques would be pertinent to assessing not only α-synuclein, but also ER-Golgi trafficking and nitrosative stress. Thus, I would conduct Western blots measure α-synuclein degradation over time, ER-Golgi traf- ficking and nitrosative stress. I would utilize antibodies against ER-Golgi trafficking markers such as carboxypeptidase-Y (CPY) for ER-Golgi traf- ficking and 3-nitrotyrosine (3-NT) for nitrosative stress (Cooper et al., 2006; Tardiff et al., 2013). Moreover, due to difficulties incurred with Western blot- ting, I would employ FM4-64 dye staining to assess the overall secretory/ endocytosis vesicular system and a nitric oxide sensor (FL2) to assess nitrosative stress (Cooper et al., 2006; Chung et al.; 2013).
Additionally, I would conduct assays investigating the possible underlying mechanisms in the mutants that drive toxicity. I would assess the amount of α-synuclein at the membrane and in the cytoplasm by puri- fying α-synuclein at both locations and conducting Western analysis. Then I would determine the level of oligomers present at the membrane and in the cytoplasm through Nuclear magnetic resonance microscopy (Ghosh et al., 2014). Next, I would assess α-synuclein structure to determine the level of α-helix and β-sheet that drives toxicity by conducting CD spectroscopy (Stöckl et al., 2013). Moreover, I would assess changes in the membrane and how they are linked to toxicity through X-ray reflectivity and atomic microscopy, which would measure the membrane properties such as thick- ness and pore formation (Hähl et al. 2014; Stöckl et al., 2013).
After measuring the occurrence of protective techniques such as sumoy- lation (Krumova et al., 2011) and overexpression of Osh2p (a yeast ho- mologue for oxysterol-binding protein) (Beh et al., 2012) in the mutants, I would attempt to rescue the cells by reinforcing these mechanisms. This way I would determine if the mutants could be rescued. Characterizing familial mutants is important, but determining rescue techniques is equally important in PD studies.
Limitations of My Study
Although there are several model organisms that may be used in the study of PD, yeast represent a powerful tool in the study of neuro- degenerative diseases as demonstrated by my study. However, my yeast model faces limitations because it does not fully model the properties of a neuron. In fact, yeast are unicellular organisms that do not naturally have the α-synuclein protein. Secondly, the neurons dying in PD are dopaminer- gic neurons that are not mimicked fully by yeast. Nevertheless, the mutants I studied require genetic manipulations that are much easier to conduct in yeast than other model organisms. Most importantly, PD is characterized by a protein folding problem that could be studied in yeast because yeast make, fold, and degrade proteins as humans do.
Importance of PD Studies
The number of PD cases is rising rapidly. Not only are PD cases increasing due to increased life expectancy, familial PD is becoming more aggressive and simultaneously decreasing the age of disease onset (Lesage et al., 2013). Therefore, studies conducted to provide insight into PD pathology are important. Although my study focused on assessing only three qualities of α-synuclein, it adds to the greater understanding of the culprit protein: α-synuclein. Understanding the mechanisms of α-synuclein in disease is important because it could lead to effective treatments and a potential cure.
Acknowledgements
First, I would like to begin by thanking Dr. Shubhik DebBurman for allowing me to join his laboratory. His support made it possible for me to begin a project that transpired into this final thesis project. I will be forever grateful for the skillset that I developed under his guidance.
Next, I would like to express my gratitude to my mentors: Madhavi Senagolage ’12, Natalie Kukulka ’13 and Katrina Campbell ’14 for teaching and guiding me as I developed my work. Furthermore, Galina Lipkin ’14, Charles Alvarado ’16 Saul Bello-Rojas ’16, Khadijah Hamid ’16, Maribel Munoz ’16, and Alex Roman ’16, my lab mates, were supportive in this process and made lab that much better each day. Additionally, I would like Elizabeth Herbert for not only providing me with additional resources, but for her guidance and love throughout the years. Also, I would like to extend a thank you to Dr. Sergio Guglielmi for always believing in me, and Dr. Douglas Light for being an invaluable resource and advisor.
Also, I would like to thank my family for providing unfaltering support. You pushed me to become better every day. Next, I would like to thank Akua Agyei, Ariane Uwamba, and Selamile Dlamini for putting up with my troubles and struggles. Thank you for your lovely friendship.
Lastly, I would like thank my committee members: Dr. Alexander Shingleton, Dr. Wentworth and Dr. Hongkyun Kim for agreeing to be on my committee and supporting me throughout my journey.